Influence of mechanical stratigraphy on deformation styles
Wiki Write-Off Entry | |
---|---|
![]() | |
Author | Zainab Alibrahim |
Affiliation | Saudi Aramco |
Competition | 2021 Middle East Wiki Write Off |
Mechanical stratigraphy is defined as the variation in mechanical properties of different rock units across the same lithostratigraphic column. These mechanical properties include tensile strength, elastic-plastic response, stiffness or competence, and susceptibility to fractures. Each mechanical stratigraphic unit is a product of chemical and mechanical changes on the rock. Despite the fact that mechanical stratigraphy is related to lithology, the description of lithology itself does not always reveal the rock mechanical properties (Fig.1).
The presence of dissimilar lithologies or structural discontinuities within a stratigraphic unit dictates mechanical stratigraphy. Both layer thickness and rigidity contrast drives variations in mechanical stratigraphy and therefore result in distinctive rock responses regardless of similarities and differences in depositional environments and conditions.
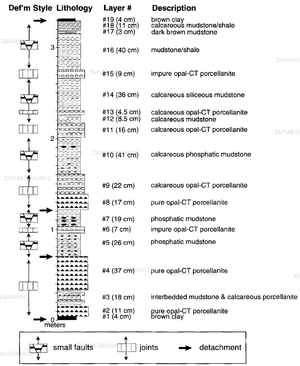
Lithological Variation Effect on Mechanical Stratigraphy
Salt Effect on Deformation Styles
Understanding the influence of mechanical stratigraphy on folds and thrust belts development and geometry requires in-depth analysis of both elastic-plastic deformation model, and contrast coefficient of adjacent rock layers. Many thrust belts structural deformation occur with the existence of certain layer arrangements consist of; weak underlying layer (shale or salt), strong middle layer (carbonate), and top cover layer (clastic) (Fig.2). This specific arrangement along with slip components enhance folding over faulting in many thrust belts deformational scenarios across the world. The high strength contrast in mechanical stratigraphy between different rock units strongly impacts the overall shape of deformation.
The presence of a salt layer can be a critical component in the evolution of structures in settings like folds and thrust belts. The deformation geometry is highly influenced by the contrast variation between weak and strong rock layers across the same stratigraphic column. Salt is known to be two times weaker than most rock types, and therefore when a décollement develops within a salt layer (i.e., horizontal gliding between two rocks) beneath an existing fold or thrust belt, salt promotes higher degree of folding. The presence of salt strongly impacts deformation style, unlike cases in when salt layer is absent. The degree to which these layer contrast may favor folding over faulting depending on the mechanical properties of the rock. For example, at high strain degree, a thick strong rock layer demonstrates high fold amplification, as strong layers tend to reach the maximum strength point faster than weak layers, and subsequently result in shortening and weakening of that layer without breaking into segments.
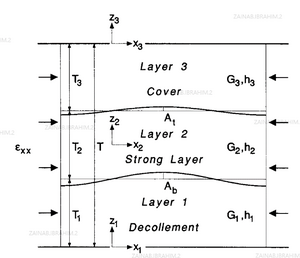
Porosity and Clay Content Effect on Mechanical Properties
Physical modeling of deformation can be useful to investigate the influence of mechanical stratigraphy and competence contrast on the evolution of structures (Fig.3). The brittle-ductile rock behavior is linked to compensation of both rigidity contrast and mechanical stratigraphy. There are multiple factors influencing variation in mechanical stratigraphy such as porosity and permeability, mineralogy, and layer thickness. The abundance of soft clay (smectite) in rock composition weakens the mechanical properties and creates rocks that are more susceptible to shear failure. High clay content accounts for strong shear strain and high concentration of faults and fractures. Moreover, high fracture intensity exists in rocks that exhibit more brittle response, while low fracture intensity is more common in ductile rocks.
Samples from the same mechanical stratigraphic unit can have different physical properties (porosity and composition). The analysis of lab experiments suggest that porosity accounts for 70% of strength variation in different lithologies while smectite content accounts for 24%. The overall rock mineralogy determines its strength. For example, 1% of smectite content reduces the rock strength by 6%, however for low porosity samples, smectite content is the main control on strength variation (Fig.4). Results indicate that fracture strength has the highest correlation with porosity and smectite content, respectively.
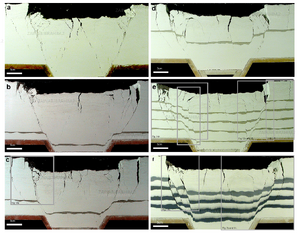
Mechanical Vs Fracture Stratigraphy
Understanding the difference between mechanical and fracture stratigraphy is critical for recognizing and predicting fracture patterns and rock behavior away from the wellbore. Mechanical stratigraphy divides rocks into different units based on mechanical properties such as brittleness, tensile strength, elastic-plastic deformation. While fracture stratigraphy divides rock units based on fracture intensity and other fracture attributes. Since fractures are controlled by rock behaviors, understanding fracture stratigraphy can reflect details about the loading history. In order to understand fracture stratigraphy, it is important to consider that the rock changes from the first fracture event until present day.
Mechanical stratigraphy changes as a result of diagenesis processes, thus both mechanical and fracture stratigraphy are not necessarily equal. Although these two terms have been used as synonyms for each other, it is not always the case that they refer to the same meaning. Mechanical properties are constantly transforming over geologic time due to diagenetic alteration, burial, and compaction processes. In other words, mechanical stratigraphy evolves with diagenesis resulting in development of fracture intensity and joint networks over time.
Different Scenarios of Mechanical and Fracture Stratigraphy
Scenario #1: Similar Mechanical and Fracture Stratigraphy
The petrophysical log has been studied for Peak Tavis Formation where fractures are a direct result of regional extension (Fig.5). Both mechanical and fracture stratigraphy are the same and can be used interchangeably. The overall fracture patterns are intense which can be predicted from the mechanical properties. Additionally, the lower part of the formation is highly fractured with low quartz cementation content, high Young’s modulus, and low Poisson’s ratio. Both mechanical and fracture stratigraphy exhibit the same patterns. For this Scenario, high fractured sandstone channels (more brittle) exhibit significant rigidity contrast with the mudstone cut banks throughout the field.
Scenario #2: Different Mechanical and Fracture Stratigraphy (Regional Loading History)
Mechanical stratigraphy and fractures are not consistent throughout the field in this case. The formation consists of interbedded marl and chalk, where chalk is more prone to fractures, however, the fracture intensity is dependent on depth and not consistent with the rest of the area. Observations like high fracture intensity at depth compared to low fracture intensity at a shallow depth were documented. (Laubach, 2009)
Dissolution, compaction, and even other digenesis alteration and precipitation seem to change mechanical properties, and therefore enhance or deteriorate fractures. Rock properties evolve with time due to mechanical and physical processes, therefore mechanical and fracture stratigraphy are different. The rock heterogeneity highly affects fracture stratigraphy and rock propensity to fracture.
The permeability is based on fracture connectivity which drives fluid flow, however, fracture intensity varies at depth and regionally. Although chalk is usually stable, recrystallization and calcite precipitation are present on a regional scale. These changes in mechanical stratigraphy are linked to clay content, and rock strength variation with no significant digenesis effect. In this case mechanical and fracture stratigraphy are not equal as deformation regionally changes mechanical stratigraphy and had affected surface and subsurface differently.
Scenario #3: Different Mechanical and Fracture Stratigraphy (Diagenetic Alteration)
During burial and complex diagenesis (involve changes in temperature) rock properties can change from ductile to brittle. In this case, lithology consists of interbedded sandstone and mudstone where later compaction took place and reduce the sandstone porosity. Mechanical stratigraphy does not match fracture stratigraphy, when a fracturing event happens prior to the present-day observed mechanical stratigraphy. The fact that mechanical stratigraphy evolved over time with diagenesis does not always result in mismatch between mechanical stratigraphy and fracture stratigraphy. The primary reason to the mismatch is that fracture event occurred before the evolution of mechanical stratigraphy, thus in this case mechanical and fracture stratigraphy are not the same
For this scenario, there are two sets of fracture, one of them cut through several layers and exhibit different strike orientations. The orientation dissimilarities suggest different fracturing events over time. Moreover, fractures that developed at deeper depth suggest alterations for different layers (sandstone and siderite), where some of these fractures are sealed while others are opened. Previously both layers had different mechanical properties before consolidation of sandstone (less prone to fractures), then as sandstone cemented, higher rigidity contrast is created and the second fracturing event took place. After cementation a new mechanical stratigraphic has evolved, however, the first set of fracture indicate the original mechanical stratigraphy.
Mechanical and fracture stratigraphy are influenced by diagenesis which alters the rock properties and fabric. Overall cementation and burial can change the mechanical properties, deformation styles, and strength or brittleness of rocks. Furthermore, linking both mechanical and fracture stratigraphy can help in fractures prediction and modeling (Fig.6).
Effect of Mechanical Stratigraphy on Joint Network
Joint propagation patterns are highly influenced by mechanical stratigraphic contrast. These patterns are used to interpret rigidity contrast and changes in mechanical properties due to digenetic alteration. The observation of joint patterns, namely bed-contained joints (an early stage), and trough-going joints (with different orientations) suggest changes in mechanical stratigraphy and existence of two fracturing events over time (Fig.7). Bed-contained joints formed when the rigidity contrast (uncemented sandstone) between beds was higher than it is today. Moreover, the integration of both outcrop observations and lab experiments on trough-going joints indicate low rigidity contrast and preservation of present-day conditions. Different stages of digenesis affect mechanical properties based on composition, grain size, and compaction. These alterations in mechanical stratigraphy affect joint networks and the overall rock behavior.
Mechanical stratigraphy can be defined by different factors which include rigidity of rock layers, relative thickness of each bed, and the interference contrast between layers. These mechanical conditions do not remain the same especially if the lithological units experience multiple fracturing events. The mechanical stratigraphy profiles change as a result of diagenetic processes developing new 3D joint networks. Understanding the joint architecture requires deep understanding of digenesis events that alter the mechanical properties of rocks.
Sometimes these joint networks act as fluid conduits and enhances the migration process for hydrocarbons, but due to the constant change in digenesis and mechanical properties, the effectiveness of these joint networks to the petroleum system can change through geologic time. Changes in mechanical stratigraphy during deformation can be studied through analyzing joint patterns. During deformation, rocks undergo different degrees of digenesis and as deformation continues, the beds containing initial joints may get rotated. Therefore these joint networks no longer preserve the original joint network orientation and properties. Two different distinct joint sets are recognized within beds intersection. The first set is within a one-bed layer and the second set is extended to different layers. The study of the Oliana anticline (south-central Spanish Pyrenees) (Fig.8) explains joint networks extended to multiple rock layers as a natural result of the rigidity contrast and variation of mechanical stratigraphy between beds (fine and very fine sandstone). Later, these variations defined how rocks deform and fracture.
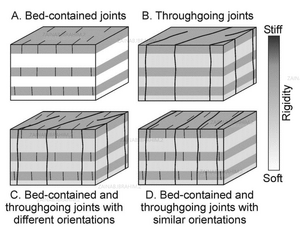
Controls on Fracture Networks
The geometry of joints is related to the lithological contrast and competency between rock layers which determine joint propagation (Fig.9). There are two main factors affecting joint propagation which include interface strength and contrast of rheology. Lab experiments show weak interface favor joint propagation, especially for soft and ductile layers. Additionally, strong rigidity contrast promotes fault termination, for example, bed-contained joint develop when (μ: shear modulus) μsoft/μStiff ratio is less than 0.4, while through-going joints appear when μsoft/μStiff ratio is greater than 0.4.
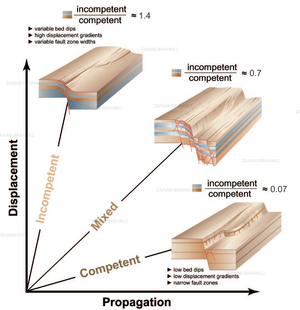
Interpretation of Changes in Mechanical Stratigraphy using Joint Pattern
The rock today preserves the recent mechanical properties and the final stages of diagenetic alteration and deformation. The rock history is investigated to understand how mechanical stratigraphy changes through time. For instance, using joint patterns can give an explanation for rheology changes. If all the joint sets are perpendicular to each and are present within one rock unit, then the contrast between beds at the time of joints initiated was relatively high.
There are cases of two different joint patterns appearing in the same rock, as the first set is contained within one bed and the other crosses different layers (interfaces). This observation suggests that two jointing events occurred where rigidity changed between these two events. Each joint pattern indicates different mechanical stratigraphic conditions based on the rigidity contrast with adjacent beds. While today’s rigidity measurements are related to the recent fracturing event can be measured through lab tests, the previous joint patterns formed under different mechanical stratigraphy conditions at a different time.
Case Study: The Oliana Anticline
Stratigraphy and Joint Patterns
The Oliana is a northeast double plunging anticline composed of different lithologies (mudstone, siltstone, sandstone, and conglomerate). The study observes outcrop exposures, the northeast limb, and joint patterns along with rock mechanics lab testing. The outcrop consists of fine siltstone and grained sandstone beds which exhibit high rigidity contrast.
The analysis indicates two joint patterns, bed-contained joins and through-going joints (Fig.10 and 11). The bed-contained set is restricted to the high resistance fine sandstone layers, while the other set shows longer joint fracture extended crossed multiple layers with dissimilar fractures orientations. This observation indicates different jointing events associated with changes in mechanical properties. The harmonic pattern of through-going joints across large scale geometry within the study area suggest it is the youngest set.
Interpretation of Joint Patterns and Rigidity Contrast
Lab test has been conducted to measure present-day rigidity using Schmidt rock hammer. Rebound data measurements and other identification of empirical rock relationships are calculated for the relative rigidity using shear modulus. Results suggest the highest rigidity contrast is between sandstone and siltstone, while the lowest contrast is between fine and very fine-grained sandstone.
The existence of both bed-contained and through-going joints in the same outcrop suggests that these two sets form at different jointing events due to changes in mechanical stratigraphy of the same rock over time. The present-day rigidity lab tests indicate contrast between beds is not too high and will not enhance more fault and joint propagation in the future. While bed-contained joints formed when the contrast between beds was high, the through-going joints formed when the contrast is low. The through-going joints are younger and resemble the mechanical conditions of present day.
The changes in mechanical conditions in the area are tied to digenetic events. Different alteration processes may increase rigidity such as dewatering, compaction, consolidation, geothermal heating, cementation, and recrystallization due to pressure solution. Also, poor lithification caused changes in rigidity contrast between layers and therefore inhibited joint propagation. Additionally, a fine sandstone layer with previously high porosity and permeability may undergo diageneses and mineral precipitation which changed the properties, resulting in a stiffer layer than before. Later burial and compaction, the sandstone may be stiffer with higher rigidity contrast allowing more through-going joint to develop.
Conclusion
Mechanical stratigraphy can evolve through time as a result of diagenetic processes and not necessarily the original depositional fabric and properties of the rock. Different alteration processes change mechanical properties for different rock types. For example, for carbonate rocks dolomitization, recrystallization, and changing from aragonite to calcite alter rigidity contrast. Other types of rocks such as clastics are affected by frost welding and thermal heating or cooling which also change rigidity and stress and strain rock response.
The Oliana anticline displays two sets of joints indicating changes in mechanical stratigraphy that can be inferred from different set orientations and other distinct features. If the two sets were formed at the same time and the same mechanical stratigraphy, they would have shown similar orientations and termination characteristics. This case study shows how different joint patterns are associated with rigidity contrast and the evolution of mechanical stratigraphy.
References
Corbett, K., Friedman, M., Spang, J., 1987, Fracture Development and Mechanical Stratigraphy of Austin Chalk, Texas, The American Association of Petroleum Geologists Bulletin
Erickson, S., 1995, Influence of Mechanical Stratigraphy on Folding Vs Faulting, Journal of Structural Geology
Ferrill, D., Morris, A., 2008, Fault Zone Deformation Controlled by Carbonate Mechanical Stratigraphy, Balcones Fault System, Texas, The American Association of Petroleum Geologists Bulletin
Gent, H., Holland, M., Urai, J., Loosveld, R., 2009, Evolution of Fault Zones in Carbonates with Mechanical Stratigraphy-Insights from Scale Models using Layered Cohesive Powder, Journal of Structural Geology
Gross, M., Alonso, G., Wacker, T., Collinsworth, K., Behl, R., 1996, Influence of Mechanical Stratigraphy and Kinematics on Fault Scaling Relations, Journal of Structural Geology
Laubach, S., Olson, J., Gross, M., 2009, Mechanical and Fracture Stratigraphy, The American Association of Petroleum Geologists Bulletin
Shackleton, J., Cooke, M., Sussman, A., 2005, Evidence for Temporal Channing Mechanical Stratigraphy and Effects on Joint-Network Architecture, Geological Society of America
Walker, L., 2003, Applied Subsurface Geological Mapping with Structural Methods, v.1, p 272.