Tools and techniques for studying mudstones
Sequence Stratigraphy: Applications to Fine-Grained Rocks | |
![]() | |
Series | Memoir |
---|---|
Chapter | Tools and Techniques for Studying Mudstones |
Author | O. R. Lazar, K. M. Bohacs, J. Schieber, J. H. S. Macquaker, T. M. Demko |
Link | Web page |
Mudstone properties vary widely, but systematically, both vertically and laterally at millimeter-to-kilometer scales. This variability can be detected by applying a range of physical, petrophysical, chemical, and paleontological methods to characterize the rocks at different scales and interpreting the resulting data using the sequence-stratigraphic approach.
This chapter concentrates on introducing the tools and techniques that provide data about texture, bedding, composition, and grain origin, which enable the characterization of mudstone strata at millimeter-to-kilometer scales. Specifically, it addresses the application of such tools and techniques to decipher depositional conditions and construct sequence-stratigraphic frameworks. This chapter provides key references for the tools and techniques commonly used to generate the texture, bedding, composition, and grain-origin data for further consideration. It then outlines our approach toward making detailed and systematic observations of the key attributes of mudstones in outcrops, cores, and thin sections. It concludes with an overview of the key sequence-stratigraphic concepts that we find useful for studying mudstones and a summary of the methods we use to construct and test a sequence-stratigraphic framework for mudstones.
What Tools and Techniques Should Be Used? Carefully define the questions and equally carefully select the tools and techniques that will give the best answers within a time—cost framework. —Potter et al.[1]
Introduction[edit]
Mudstones are important sources, reservoirs, and seals of hydrocarbons and dominate the sedimentary record[1][2][3][4][5][6][7][8]. Mudstone properties vary systematically—both vertically and laterally at millimeter-to-kilometer scales. This variability is controlled by the changes in the depositional environment and burial history, and is recorded in the stratal stacking[9][10][11][12][13][14][15][16][17][18][19][20][21]. Sequence stratigraphy provides a genetic framework within which the varying source, reservoir, and seal attributes of mudstones can be predicted. The attributes of mudstones that need to be recorded to utilize this method are their (1) texture (grain size), (2) bedding, (3) composition, and (4) grain origin (see Mudstone nomenclature). Information regarding these attributes reveals essential aspects of the processes that form, transport, deposit, and then modify the component grains and their associated pore spaces in all depositional and burial environments.
Tools[edit]
This section covers visual, geochemical, well-log, and seismic tools and techniques. Visual tools include optical inspection, digital imaging, and optical and electron microscopy. Geochemical tools comprise bulk, elemental, and molecular techniques. Well-log tools include techniques for estimating lithology, porosity, pressure, hydrocarbon presence and type, and structural characteristics (fractures, faults, folds, in situ stress). Seismic tools cover seismic stratigraphy and facies analyses and mapping.
Visual Tools[edit]
You can observe a lot by just looking. —Yogi Berra[22]
Careful visual observation at the macro-to-microscale is the essential first step in characterizing mudstones, selecting appropriate samples for additional analyses, and deciphering the depositional conditions. We recommend that mudstone be examined visually at a millimeter-to-centimeter scale in outcrops, cores, and thin sections to determine texture, bedding, and composition, as well as physical sedimentary structures; type, diversity, and abundance of body and trace fossils; bioturbation index; taphonomy of macrofossils; type, habit, and distribution of diagenetic products; fractures; and color[20][21][23].
Visual observations should be made on surfaces of fresh rock in outcrops and cores (Figure 1), and then supplemented with observations made using tools that include high-intensity white and ultraviolet light, and optical, electronic, and digital imaging of hand specimens and thin sections. Observations should include the abundance of each physical, biological, and chemical attribute to assist in the identification of facies, facies associations, and associated stacking patterns[17][20][21]. Visual observations can be facilitated and enhanced by using the tools and approaches that are discussed next.
Preparation of Hand Specimens and Core Slabs[edit]
A clean, dry rock surface is an essential prerequisite to any form of visual examination. The time spent preparing fine-grained rocks is amply rewarded by the many details revealed (Figure 1). A list of tools and supplies we recommend for preparing fresh rock surfaces and describing mudstones in outcrops and cores is given in Table 1. We recommend the following tools and approaches for preparing mudstone surfaces for visual examination:
Table 1. Suggested List of Equipment and Supplies for Describing Mudstones (after Lazar et al.[21]).
- Backpack
- Geologic hammer
- Compass
- Jacob staff
- Magnifiers:
- Hand lens: 10×, 20×
- Pocket microscope: 25×, 50×
- Binocular microscope, reflected light*
- Portable lamps (white and UV light)*
- Pocket scratcher (sharp steel probe)
- Acid bottle (10% HCl)
- Water spray bottles1
- Grain-size chart
- Towel paper, bucket, sponges1
- Brushes, chisels
- Penknife/multitool
- Tape, ruler
- Sample bags
- Pencils, markers
- Eraser1
- Field notebooks
- Clipboard or clipboard case (letter size)
- Digital camera
- Laptop
- White multiuse ¾"× 1" labels to mark the depth of samples and relevant details to be photographed (include core name and depth) 1
- Color Post-it flags or notes to mark significant candidate sequence-stratigraphic surfaces (red—sequence boundaries; green—maximum flooding surfaces; blue—transgressive surfaces and parasequence boundaries) 1
- Whistle, flashlight, small first aid kit
1supplemental gear for core description.
- Sharp-rock saw: Prepare a flat, relatively polished surface. A portable concrete saw can be used in the field to acquire continuous, perpendicular-to-bedding slot samples; samples should be stabilized with fiberglass matting and epoxy resin prior to removal[12].
- Clean: Scrub and polish using a paint brush, scrub brush, abrasive paper or sponge (polished using a series of sandpaper of grades from no. 80 to no. 800 grit); scrubbing pad (softer—white and harder—green); or pencil eraser (∼1 in. wide, pink or white). Pencil erasers can be quite effective for removing dust, precipitated minerals, or oil-based mud from slabbed-core pieces. Beware of rocks that are sensitive to water—minimize exposure time and dry each piece immediately, using compressed air, if possible, or a clean absorbent material (e.g., paper towel). Use a dilute degreasing solution on cores taken with oil-based mud.
- Contrast enhancement techniques: Several approaches are available that are fast, simple, and useful for visualizing sedimentary details if further geochemical analyses of the studied rocks are not planned. Many of these approaches are summarized in the literature[24][25][26][27]; some of these approaches are:
- Etching in dilute hydrochloric acid[27]: excellent for enhancing texture.
- Wetting with water[28]: excellent for emphasizing bioturbation.
- Staining with an ink: Alizarin Red S[29][30], a mixture of India ink and carborundum powder[31], and periodic acid–Schiff[32]; excellent for identifying composition and porosity[27][33][34][35].
- Modified Bushinsky oil technique[36][37]: excellent for enhancing the visibility of internal structures and details of fabric in chalk. Samples are slabbed, polished, and then painted with lightweight oil.
Light Sources[edit]
A strong light source is an essential tool for making visual observations in mudstones. The source, intensity, and angle of illumination are all important factors. Useful light sources include the following:
- White light: Sunlight is best, but it is not always available. Remember that the intensity of light changes during the day. We recommend a broad-spectrum, artificial light source that provides an even intensity across the examined sample (at least 10–15 cm across). Several models of battery-operated or AC-power–supplied lights with multiple light-emitting diodes (LEDs) in linear or circular arrays are available.
- Ultraviolet (UV) light: We recommend using several wavelengths of ultraviolet (UV) light to enhance different aspects:
- Longer wavelengths (∼365 nm/UV-A) enhance detection of hydrocarbons and various types of organic matter (e.g., spores, cutin, resins, waxes, fats, algal cysts, and bacterial degradation products[18][38].
- Shorter wavelengths (∼254 nm) enhance visualization of mineral species.
- It is helpful to construct an illumination and viewing bench suitable for examining slabbed-core samples[18].
- Eye and skin protection are essential when using UV light sources.
Optical Imaging[edit]
Handheld magnifiers are useful for determining the grain-size distribution and details of bedding, body, and trace fossils. These tools include the following:
- ‘’Hand lens:’’ Typically, the resolution of the naked eye is no more than 100 μm. The most useful magnifications are 10x and 20x; a 10x hand lens can resolve down to 10 μm, and a 20x hand lens can resolve down to 5 μm under optimal conditions. A good magnifier has a moderately large field of view and flat image, with minimal spherical aberration.
- ‘’Pocket microscope:’’ A 50x pocket microscope, with attached light, is also useful, especially for a quick identification and description of microfossils and small nodules.
- ‘’’Digital handheld microscope:’’ These units come with a variety of magnifications and focal distances and are able to capture images with scale bars. Some can broadcast images via Wi-Fi to tablet computers and smartphones, which are very useful when working with large groups.
Digital Imaging[edit]
Digital images are useful for both revealing subtle aspects of the rocks that might not be readily apparent to the naked eye and documenting the observations made. These images can be acquired in several ways:
- ‘’Camera system’’: A camera system includes the camera body, lens, and lighting. All the three aspects need to be optimized for capturing useful images of mudstones:
- ‘’Camera body’’: Of especial importance are the sensor type and configuration, the megapixel range, and the construction (rugged, durable, dust and weather resistant, compact but easy to use).
- ‘’Lens’’: A macro lens is very useful for close-up photography. It is specifically designed to provide maximum resolution and sharpness with minimum distortion at its closest focus. Macro lenses come in various fixed focal lengths (typically 50–200 mm). Shorter focal lengths have a slightly greater depth of field but closer working distances that can make lighting a challenge. Longer focal lengths allow more flexibility in lighting but with lesser depth of field. We recommend a lens at the long end of standard macro lenses (between 100 and 105 mm) as it provides a comfortable working distance (9–12 in. [20–30 cm]) with an acceptable depth of field. Several compact digital cameras have quite good macro capability (part of this capability is inherent in the small lens and sensor size), but it is essential to test the actual model on typical subjects to determine which one is most appropriate for your intended uses. Lens filters might be necessary to allow artificial colors or change in light intensity.
- ‘’Lighting’’: Achieving even illumination of the subject at close working distances is a challenge in photographing mudstones. Sufficient intensity is also necessary to allow short exposure times at small f-stops—this leads to maximal sharpness and depth of field. For shorter focal length lenses, we recommend using a ring flash or ring light that mounts directly around the lens and provides illumination that can be varied in intensity and ratio. A normal flash unit that connects to the top of the camera can be used, but the camera must be at least a meter or more away from the subject—this necessitates a longer focal length lens to fill the frame with a small subject.
- ‘’Accessories’’: Depending on the type of sample and the amount of photography to be done, a copy stand or a tripod setup, large memory capability for photo storage, and wireless remote triggers are helpful.
- ‘’Digital scanner’’: A flatbed scanner is a fast and easy-to-use imaging tool for an object that has a flat surface and is relatively small (e.g., a piece of slabbed core or a thin section).
- ‘’Flatbed scanner’’: We recommend a flatbed scanner with a range of optical resolution capabilities (300–6400 dpi), high maximum density (4.0 Dmax), 48-bit color and 16-bit gray-scale output, dust/scratch removal, and sharpness capabilities.
- ‘’Fluid-immersion scanning’’ can be helpful for certain types of mudstones:
- As with optical microscopy, fluid immersion increases the resolution of the lens, and that enables the lens to achieve higher magnifications. (The numerical aperture of the lens is proportional to the refractive index of the fluid.)
- For fluid-immersion scanning, the slabbed sample is immersed using a glass-bottomed tray specially designed for the scanner used, with fluid between the glass and the slabbed face. The benefits of this method include reducing losses through reflection and scattering (making the image appear brighter and with greater color saturation) and increasing transmission integrity (providing better definition of shadowed areas and greater dynamic range).
- The usual considerations and precautions must be taken with selecting and using a fluid that does not adversely affect the rocks being scanned.
Image Processing[edit]
Image manipulation of hand specimens, cores, and thin sections using Adobe PhotoshopTM or similar software can reveal the presence of subtle sedimentary features and enable estimation of the degree of compaction of the studied mudstone[39][40]. For example, image enhancement with image processing software (following manipulation of autolevels, brightness, contrast, color balance, and saturation) can reveal subtle discontinuities and disruptions of laminae and subtle burrows in mudstones with very little variation in color, texture, and composition[40]. These software applications can also be used to produce an image that approximates the rock’s precompaction state. The steps we recommend for achieving this “virtual decompaction” are as follows:
- Select perpendicular cuts of horizontal burrows.
- Resize image by holding the horizontal dimension constant and changing the vertical dimension stepwise until the selected burrows show circular cross sections[39].
Microscopy[edit]
By the help of Microscopes, there is nothing so small as to escape our inquiry; hence there is a new visible World discovered to the understanding. —Robert Hooke[41]
Examination of rock samples and properly prepared thin sections is an essential early step in characterizing mudstones, specifically providing information about grain size and composition, as well as insights into the depositional and diagenetic processes. Because mudstones are generally rather soft, preparation of good thin sections can pose a challenge. Various methods of thin-section preparation, using special epoxy resins, multiple epoxy impregnation, diamond laps instead of loose grit, double polish, and so on, have been developed[25][42][43][44] [45]. Critical to remember is that mudstones are highly variable in composition and cementation history, and thus, the preparation methodology has to be fine-tuned to the specific features of a given rock. A set of tips for thin-section preparation are given later in this section.
For a good number of years, only a relatively small number of individuals made a sustained effort to communicate the benefits of thin-section petrography in mudstones[11][12][13][14][46][47][48][49][50][51][52][53][54][55]. The recent boom in hydrocarbon production from these rocks, however, has brought the general attention to the complexity of mudstones and the significance of observations made under the microscope. As a consequence, studying thin sections of mudstones has become significant as shown by a growing number of related recent publications[56][57][58][59][60][61][62]. Examination of thin sections under the microscope is, in our opinion, a crucial source of information on sedimentary features, mineralogy, diagenesis, and microfossil content, and should be performed early on in any investigation of a mudstone succession.
Thin Sections[edit]
Evaluations of primary rock attributes described directly in outcrops and cores can be enhanced by integration with observations made in thin sections. Examination of polished thin sections can be performed under a petrographic microscope and, if available, a scanning electron microscope (SEM). Examination of thin sections is particularly useful for identifying the presence of composite grains (e.g., floccules, faecal pellets, and intraclasts) and recognizing the effects of cementation, dissolution, and bioturbation on grain size[20][21]. Examination of large, polished thin sections allows for imaging and investigating of multiple genetic beds[20][21]. Additionally, examination of polished thin sections is particularly useful in distinguishing the composition of individual grains and cement, particularly using an SEM equipped with combined energy-dispersive, cathodoluminescence, and backscattered electron detectors, as discussed in the following sections[13][14][52][60][54][63][64][65][66][67][68].
We recommend this workflow for the process from mudstone sampling to preparation and examination of thin sections:
- Obtain representative samples of all facies identified in the core or outcrop (see Mudstone tools and techniques#Sample Selection—General Considerations section and Lazar et al.[21]for guidelines for mudstone sampling). Wrap outcrop samples in aluminum foil and tape to prevent rock breakage and excessive development of mold during sample transportation.
- We recommend that thin sections be prepared early in the investigation. If there are no sample-size limitations, it is particularly valuable to make large, polished thin sections (76 mm x 48 mm [∼3 in. x 2 in.]).
- We recommend that, after a quick examination under the microscope, and depending on rock texture and composition, “ultra-thin” sections (<20 μm) be prepared to maximize the textural information that can be obtained from the examined mudstones[21][54][69][70]. Many constituent particles in mudstones tend to be smaller than the 30 μm thickness of a standard thin section, and thus, larger grains (coarse silt and larger) are more conspicuous under the microscope. In contrast, particles that measure in the 5–30 μm size range become “invisible” within the thin “slab” of material that has been sectioned, especially when encased in a matrix that does not provide much contrast. To counteract this problem, one can resort to the manufacture of “ultra-thin” sections or simply take advantage of the fact that many thin sections develop “wedged” margins while thinned to the final thickness. Simply examining the wedged part allows one to view particles that are much smaller than the thickness of the standard thin section. Double-polished thin sections can also be helpful because grain relations are more readily observed when surface roughness on both sides of the “slab” has been minimized. (Grain size is a fundamental attribute of sedimentary rocks. See, for example, McCave and Syvitski[71] for a review of principles and other methods of particle-size analysis. Interpretation of results obtained by such methods should be made with caution, bearing in mind that mudstone samples may be disaggregated to particle sizes that are actually not present in the rock—and reveals more about the disaggregation process rather than the original grain-size distribution.)
- Polished thin sections have multiple benefits: They do not only improve the visibility of features for transmitted light microscopy but also enable reflected light microscopy, fluorescence microscopy, cathodoluminescence (CL) microscopy, scanning electron microscopy, and electron microprobe analysis.
- Preparing polished thin sections of mudstones is labor intensive because constituent minerals have a wide range of hardness (clay minerals are soft, and cementation may be absent or variably developed), and it is difficult to inject stabilizing epoxy into the tiny pores of these rocks. Samples can also be damaged during the preparation of thin sections because mudstones commonly contain relatively high proportions of hydratable clay minerals. To minimize sample damage, argillaceous mudstones should be exposed to as little water as possible during the preparation process[21]. Thin-section petrography of soils deals with similar aspects as thin-section petrography of mudstones, and thus, the handbook for soil thin-section description by Bullock et al.[72] is a handy and compact supplement to other texts on thin-section preparation and description.
- Scan the thin sections on a flatbed, high-resolution scanner to produce high-quality, low-magnification images that enable a detailed study of the physical, biological, and chemical sedimentary features present.
- Capture the relevant features with a digital camera attached to a petrographic microscope.
- Use image processing software (e.g., Adobe PhotoshopTM) to bring out seemingly obscure features (image enhancements: levels, brightness, contrast, color balance, sharpening, etc.).
We recommend documenting thin-section observations of texture, bedding, and composition using a fit-for-purpose template (see Lazar et al.[21] for template examples). Thin-section templates should enable one to record observations made on key attributes of the mudstones and should be adapted to the objectives of and the time available for a specific project.
Optical Microscope[edit]
Optical microscopy can, for example, inform about the not-always-so-obvious origin of quartz grains[64][73][74], the formation history of small spots of cherty-looking material[56], depositional parameters[13][20][21][55][75], and sequence-stratigraphic packaging and parasequence stacking patterns[18][20][21][76]. For example, Schieber[73] was able to show that diagenetic infilling of algal cysts produced sand-size diagenetic quartz grains that are easily mistaken for detrital grains, which can lead to erroneous interpretations of mudstone-associated sandstone beds. This avenue of research was extended further when in distal mudstones, large proportions of silt-size (and presumably detrital) quartz grains were linked to early diagenetic processes as well[65]. Chertlike grains may initially suggest a diagenetic origin, however, these grains can also be detrital with some help from grain-concentrating benthic agglutinated foraminifera[51][56].
When examined closely, many mudstone successions also show a wide variety of primary sedimentary structures and bioturbation features at thin-section scale and can provide excellent clues to sedimentary conditions, such as the presence of bottom currents[13][77], event deposition[49][13][78][79] and microbial mats[13][49], as well as to substrate consistency[39][80] and more subtle forms of animal–sediment interaction[40][81]. See Mudstone nomenclature, and Laminasets, beds, and bedsets, as well as all of the case study chapters (Bohacs and Ferrin[82], Bohacs and Grabowski[83]; Bohacs and Guthrie[84]; Bohacs et al[85][86], Campo et al.[87]; Lazar and Schieber[88]; Potma et al.[89] for other representative examples.
Another microscopic approach to mudstone petrography is the examination of silt- and sand-size constituents in grain mounts after separation from the mudstone matrix. This furnishes additional information on the provenance of the fine-grained sediment and the diagenetic alteration of grains. Separation of this grain population by means of heavy liquids or magnetic separators or both allows a further split into light and heavy minerals, and facilitates the study of rare constituents that are not manifest in conventional thin sections. Auxiliary methods for analysis are an SEM with an attached energy-dispersive x-ray analysis system (EDS) or an electron microprobe (discussed in “Elemental Mapping” paragraphs in the “Scanning Electron Microscope” section).
Thin-section examination under either the optical microscope or the SEM has also been used to detect grain-size trends in stratigraphic successions[55], but some caution is advisable in that regard. Grains smaller than section thickness tend to be less readily observed, and grains larger than section thickness will not only be more conspicuous, but their observed average size will be smaller than the actual size because few will be sectioned through the widest part of the grain. Thus, trends from sand to silt can probably be determined with reasonable assurance, whereas trends within, say, the silt range are difficult to reproduce consistently. One could, of course, also try to measure grain-size trends via settling-tube analysis from the disaggregated samples, but there the problem is that it is nearly impossible to disaggregate a lithified mudstone into its component grains. As such, results of this kind of analysis need to be evaluated critically, unless the studied rock is indeed very poorly consolidated.
Scanning Electron Microscope[edit]
The scanning electron microscope (SEM) has long been a powerful tool in its various applications to sedimentary rocks[46][90][91] and has proven indispensable for the investigation of mineral relations and textures in fine-grained rocks[92]. Examination under an electron microscope provides useful insights into grain composition and origin as well as depositional processes and diagenetic history[53][60][65][73][93]. Composition-content SEM studies have revealed decameter-scale stratigraphic patterns that assisted sequence-stratigraphic interpretations[55][94].
Fundamentally, ‘SEM imaging’ relies on secondary electron emission that is sensitive to the surface relief of the sample as well as to compositional differences when operated in the backscattered electron mode. Several chapters in AAPG Memoir 102 introduce up-to-date operation principles and sample preparation methods[95][96].
Although SEM magnifications can range from single digits to more than 100,000x, it is the 1000x–10,000x range that is commonly used for textural studies, whereas the 20,000x–120,000x range is commonly used for studies of porosity and detailed mineral interrelations. Because of its great depth of focus (compared with petrographic microscopes), the SEM allows detailed imaging of rough and broken surfaces (e.g., drill cuttings), but its power as a petrographic tool really comes to the forefront when flat surfaces are examined.
SEM analyses require high-quality polished surfaces for reliable results. Although such flat surfaces have traditionally been prepared by mechanical grinding with successively finer polishing media (polished thin sections), the associated mechanical damage manifests itself via surface “smear” and obscured details of mineral relations and pore spaces. The more recent application of ‘argon ion milling’, a sample preparation technique developed by material scientists[97], overcame this limitation and enabled imaging of pore spaces and mineral interrelations at the magnifications needed for mudstone studies[50][98][99][100][101][102]. Pores are readily observed in transmission electron microscopy (TEM) images of argon-ion-milled samples[99], but today the preferred technique for imaging pores in mudstones is the SEM examination of argon-ion-milled surfaces[52][93][103][104][105][106][107][108]. The potential for generating artificial pores in organic-material-rich areas resulting from ion beam heating has been a serious concern. See Schieber et al. [108] for a discussion about a procedure to eliminate ion-milling artifacts.
SEM analyses can be used for ‘elemental mapping’—One of the very useful by-products of electron beam imaging is the emission of x-rays from the surface of a sample. Because each chemical element has a unique atomic structure, it produces a unique set of characteristic x-rays that can be differentiated with an x-ray spectrometer. In SEM applications, energy-dispersive x-ray spectroscopy (commonly abbreviated as EDS or EDX) is the most widely employed method, although wavelength-dispersive spectroscopy (WDS; used in electron microprobes) is superior for quantitative analysis. Within the resolution limits of modern EDS detectors (about 0.2–1 μ), each image spot that is scanned with an SEM can also be associated with an EDS spectrum that carries information about the chemical composition of that spot[109]. Software manipulation of spectral datasets can be used to extract maps for chemical elements of interest and also to map the object classes (e.g., minerals) within the field of view. These element maps are very useful for the identification of minerals and for estimates of rock composition.
Another useful by-product of electron beam imaging is ‘cathodoluminescence’. Cathodoluminescence (CL) is the photoelectric effect in reverse. Electrons interact with a luminescent material (usually nonconductive or semiconductive) and cause emission of photons in the wavelengths of the visible light spectrum (as well as beyond: infrared and ultraviolet). CL is observable by petrographic microscope (floodgun) or by SEM where other detectors can support mineral identification[110][111]. Rock-forming minerals (e.g., quartz, feldspar, carbonate, and apatite) can be identified on the basis of their CL colors[110][112][113]. A drawback of the “floodgun” approach is that high densities of energetic electrons can induce undesirable high specimen temperatures, cause reduced CL emissions, and even cause permanent damage to the CL emission of the specimen. In contrast, scanning for CL in the electron microscope (SEM-CL) gives much higher spatial resolution, and because the beam “rests” on a given spot for only a few microseconds, heating effects are greatly reduced. State-of-the-art detector designs, such as the Gatan ChromaCL, enable live digital color imaging at a range of pixel resolutions. All CL information is acquired in a single imaging pass, and potential beam damage to CL-related features is greatly reduced.
Cathodoluminescence emissions are dependent on several factors, including but not limited to crystal structure, lattice defects, and distribution of trace or minor elements. As a tool for detection of chemical differences, CL is, in most cases, much more sensitive than EDS and can allow visualization of differences in the ppm concentration range. By showing the distribution of trace and minor elements, as well as crystal growth patterns, SEM-CL can be a very valuable petrographic technique that is able to show features that are undetectable with other techniques.
Another application where CL imaging has the potential to add to the understanding of sedimentary successions is in the determination of sediment provenance[114]. The CL color (or spectrum) of detrital quartz grains reflects the rocks from which the quartz originated. This was first comprehensively documented in an early study by Zinkernagel[113] and has been confirmed by subsequent studies. Traditionally, provenance assessments of mudstones used bulk rock geochemical parameters, which are prone to bias by weathering and diagenesis. Thus, for a long time, mudstones have been underused for provenance studies, although they constitute the bulk of most sedimentary basin fills. With time, increasingly more studies of CL textures for quartz sourced from a variety of igneous, metamorphic, and sedimentary rocks have been conducted[63]. The new generation of color CL detectors (like ChromaCL2TM) makes it possible to obtain good-quality provenance information from large quartz grains down to those in the silt-size range by identifying populations of quartz silt grains of similar provenance and diagenetic history through the combined use of the CL color and grain internal textures[115][116] [117]. Applying SEM-CL to quartz grains, the chemically and mechanically most robust major component of these rocks, will in the future enable us to greatly improve our understanding of provenance and uplift history of the source area of sedimentary basin fills.
X-Ray Computed Tomography Scan[edit]
X-ray CT (computed tomography) examination is most useful for 3-D visualization of bedding and (subtly expressed) bioturbation; it can also provide useful insights into grain-size distribution and composition (organic-rich or cemented strata). Information on depositional conditions comes from interpreting the lamina geometries and bedding styles, which can be challenging to resolve with normal optical means. For more information on this method, see Ketchum and Carlson[118], Spaw et al.[119], Ketchum and Hildebrandt[120], and Spaw[121]
Sample Preparation and Analytical Considerations[edit]
- X-ray CT scans are rapid and nondestructive; they reveal the 3-D internal bedding and properties of mudstones through directing x-rays at a core from multiple angles and measuring the energy transmitted through the core. The images obtained are compiled into video formats and record variations in x-ray attenuation by the core material, which is a function of the density and composition (atomic number) of the rocks (as well as the incident x-ray energy and path length of the x-ray beam).
- This analysis is best suited for cylindrical samples. The only length limitation is the capacity of the machine.
- It can be done on slabbed or whole core (in or out of the liner/sleeve).
CT Data Interpretation[edit]
- CT data are captured in a succession of image files suitable for visualization and analysis using various 2-D and 3-D image processing tools.
- Standard modes of 3-D visualization include volume rendering and isosurfacing. Volume rendering is commonly the most useful for sequence-stratigraphic analysis.
Tips and Traps[edit]
- CT scans are best acquired when the core is intact because artifacts from broken core pieces and imaging procedures can complicate interpretations.
- CT scans work best on mudstones with relatively high contrast among their components (e.g., clay minerals, carbonate, or pyrite). It would, however, be difficult to differentiate carbonate shells in a fine-grained carbonate matrix.
- Resolution is typically limited to 1000–2000 times the object’s cross-sectional diameter (∼50–100 μ for a typical 4 in. core).
- Each CT scan produces multiple gigabytes of data and requires significant computing resources for manipulation, visualization, and analysis.
Procedures to Capture Mudstone Description in Cores and Outcrops[edit]
Millimeter-to-centimeter-scale observations of mudstone strata and their stacking patterns are recorded keeping track of the abundance of each physical, biological, and chemical attribute. One can then recognize laminae, laminasets, beds, and bedsets, and aggregate these observations into facies, facies associations, and facies-association successions[17][20][21]. An example of a description form and symbols we use to capture lamina-to-bedset-scale observations in mudstone successions is given in Figure 2. Such a form clearly separates observations from interpretations, along with differentiating the various levels of interpretations; it captures observations on the left side; first to the right are lower level interpretations (e.g., sedimentary structures) and then higher level interpretations (e.g., benthic-energy and oxygen levels, depositional environments; Figure 2).
[[file:M126CH3-Figure2.jpeg|thumb|300px|Figure 2 2A. Example of a form that we recommend using to capture the key attributes of mudstones observed in cores. 2B. Example of symbols useful for capturing mudstone observations in cores and hand specimens (after Lazar et al. [21] used with permission).
Observations[edit]
Key attributes to capture include the following (see also Table 2 and Mudstone nomenclature and Laminasets, beds, and bedsets:
- Depth or elevation
- Sample or photograph unique identifier
- Grain size: minimum, mode, maximum
- Lamina geometry: continuity, shape, geometry (figure 1B of Mudstone nomenclature)
- Bedding surfaces: location, shape, spacing
- Composition: argillaceous, siliceous, and calcareous (figure 1C of Mudstone nomenclature)
- Physical sedimentary structures: primary, secondary
- Biogenic material: type, abundance, diversity, and taphonomy (figure 1F, G of Mudstone nomenclature)
- Ichnofauna: type, diversity, and abundance
- Diagenetic products: nodules, cement
- Bioturbation index (figure 1E of Mudstone nomenclature)
- Fractures: type, abundance, orientation, mineralization
Interpretations[edit]
It is important to comment on the following (see also Table 2 , Laminasets, beds, and bedsets, and Parasequences[122]):
- Origin of grains
- Transportation, depositional, and reworking processes
- Diagenetic processes
- Environment of deposition
- Sequence stratigraphy: surfaces, stratal units
Comparison Charts[edit]
Consistent capture of hand specimen and core observations is facilitated by using various charts for estimating grain size, percentage of a particular grain type, degree of bioturbation, or color. A brief introduction to these charts follows:
Grain-Size Charts[edit]
These charts classify sediments and sedimentary rocks based on the range of the diameter of individual grains[123][124][125]. See Mudstone nomenclature for a discussion and definitions of mud grain-size boundaries. As a reminder, before examination of mudstone strata, it is important to obtain and examine fresh surfaces of rocks from each potential stratigraphic package. Then, estimate the percentage of sand-size grains with visual comparison to percentage charts. If less than 50 % are sand-sized grains, the rock is a mudstone and next, a scratch test can be performed to determine the dominant grain sizeCite error: Closing </ref>
missing for <ref>
tag, small and large angular grains[126], spherical grains[127], irregularly shaped grains of various shapes[128], or nearly spherical (crinoidal) or blady (pelecypod) skeletal grains[127].
Bioturbation-Index Estimator[edit]
The degree of bioturbation can be assessed using a 0–5 scale[20][21] (figure 1E of Mudstone nomenclature). Bioturbation tends to be subtle in mudstone strata; do not expect too many textbook-style ichnofossils because burrows tend to be obscure where matrix and fill do not differ in composition and rheology[21]. Prior to mud compaction, benthos do not burrow but swim through muddy sediment with high water content (70%–90%) and disturb the sediment fabric, producing deformational structuresCite error: Closing </ref>
missing for <ref>
tag, Pemberton et al.[129][81], Droser and Bottjer[130][131][132][133], Bottjer et al.[134], Bottjer and Droser[135], Schieber[40], Hasiotis[136], and Gerard and Bromley[137].
Consistency of Marine Substrate[edit]
Organisms bioturbating fine-grained sediments may produce different burrows depending on substrate consistencyCite error: Closing </ref>
missing for <ref>
tag[138][139]. Five levels of substrate consistency can be inferred from ichnofossil analysis—from “soupground” to “hardground” ().
Rock-Color Charts[edit]
A rock-color chart is useful in describing the colors of mudstones. The colors of mudstones can be compared to the Geological Society of America or Munsell color charts. The color of mudstones varies significantly and may be in various shades of black, gray, green, or brown.
- It is important to record the time of day that a mudstone description was made, as well as the light type and intensity, and the wetness state (dry or wet).
- Composition, especially the amount of iron (and its oxidation state) and organic-carbon content, is the main factor that determines mudstone color[1][21][70][140]. Organic-carbon-rich rocks are typically dark chocolate brown (5YR3/4–5YR2/1). Gray or green mudstones are typically organic-carbon lean. Mudstones with a high content of disseminated pyrite are typically grayish black to black[21] (N2–N1).
- Weathering and diagenetic processes can significantly alter mudstone color.
Geochemical Tools[edit]
Chemistry: that most excellent child of intellect and art. —Sir Cyril Norman Hinshelwood[141]
Numerous molecular, elemental, and isotopic tools that are useful for characterizing mudstones are available. These geochemical tools range in use from a quick screening (e.g., reaction to hydrochloric acid) to extremely refined estimates of microbial respiratory pathways (e.g., compound-specific isotopic analysis). Listed in this section are tools that are either commonly used by a geologist during initial mudstone investigations or available later to augment and expand the understanding of mudstone character and deciphering depositional conditions under which these rocks formed. Many other analytical tools are used for characterizing mudstones and inferring depositional conditions. For example, such analytical tools can be used to determine total uranium concentrations (delayed neutron counting) or base metal content, and trace and rare earth elements (inductively coupled plasma mass spectrometer or inductively coupled plasma atomic emission spectroscopy).
Sample Selection—General Considerations[edit]
As with all methods that analyze a very small volume of rock, it is essential to select representative samples within a geologic-stratigraphic context that enables interpretation and application (upscaling) of analytical results appropriately. Spot-sample analyses provide insights into mudstone character and depositional conditions. Profiles of analytical results for successions of samples are required for sequence-stratigraphic analyses. In either case, a sampling strategy that considers geological context and project goals is essential. Special care must be exercised in selecting samples for vertical profiles to allow robust conclusions to be drawn—samples need to be taken from equivalent facies or locations with respect to significant stratigraphic surfaces (e.g., parasequence boundaries) to discern secular trends accurately. Some of these concerns are mitigated with analytical tools that provide a near-continuous record (mm-to-cm scale), such as an x-ray fluorescence (XRF) scanner. See Lazar et al.[21] for more detail on sampling strategies.
Reaction to Hydrochloric Acid[edit]
Carbonate minerals effervesce to various degrees when exposed to dilute hydrochloric (HCl) acid. The onset, extent, and rate of effervescence are influenced by mineralogy, amount, and particle size, along with the temperature of the sample and the temperature and concentration of HCl[142]. Carbonate minerals are an important component of mudstones that attest to rock character and depositional conditions. Carbonate minerals can be of biogenic, detrital, or diagenetic origin. The acid test is useful for making a first approximation of carbonate content and mineralogy, but further work is required to reveal its origin. The composition of early-formed cements and nodules in mudstones is a function of depositional conditions, and certain compositions are associated with specific types of sequence-stratigraphic surfaces. For example, calcite or calcite and pyrite are common below parasequence boundaries, dolomite with maximum flooding surfaces, and siderite with sequence boundaries or other bypass surfaces (see discussions in Parasequences and Parasequence sets and depositional sequences[143]). For safety notes and more information on the acid test, see the report by the Soil Survey Division[142].
Standard Procedure[edit]
- Obtain a fresh surface of a mudstone sample.
- Scratch the fresh surface of the mudstone sample with a sharp steel probe.
- Apply a drop of dilute HCl onto the fresh surface and powder.
- Observe and rate reaction:
- Instant, vigorous effervescence of fresh surface indicates calcite.
- Rapid, strong effervescence of powder (and slow, weak effervescence of fresh surface) indicates dolomite or siderite.
- Siderite can sometimes be differentiated from dolomite as it tends to weather bright orange to red-brown.
Tips and Traps[edit]
- Standard concentration of HCl for this method is 1 M.
- The degree of effervescence can be classified using a table such as the one found in the report by the Soil Survey Division[142].
- The degree of effervescence is influenced by the temperature of the sample and the temperature and concentration of HCl.
- Hydrochloric acid is corrosive. Always protect the eyes and skin when working with concentrated acids.
Staining for Mineral Identification[edit]
Rapid identification of some carbonate minerals can be done using stains[33][144][145]. Stains are inexpensive, easy to use, rapid to apply, and quite versatile as they can be used in the field, on rock slabs or thin sections. Stains work by reactions whose occurrence and extent are influenced by mineral composition and crystallography. Of especial interest for sequence-stratigraphic analyses and insights into depositional conditions is the potential to differentiate aragonite, calcite, ferroan dolomite, and siderite. For more information, see Friedman[33], Evamy[34][146], Dickson[147][148], and Friedman et al.[145]
Common Procedures[edit]
- The most widely used stain is a mixture of Alizarin Red S (ARS) and potassium ferrocyanide (PF) in dilute HCl for 1–2 min. It differentiates calcite and aragonite from dolomite and siderite.
- For samples with low iron concentrations, a dual staining procedure can be used: PF + HCl, followed by ARS + PF stains as above.
- Aragonite can sometimes be distinguished from calcite using Feigl’s stain. See Friedman[33] and Schneidermann and Sandberg[149] for more information.
Tips and Traps[edit]
- The occurrence and extent of staining is a function of the reactivity of carbonate mineral species with the acid, so the crystal size and temperature of the sample, and the temperature and concentration of the acid influence the outcome.
- Hydrochloric acid is corrosive. Always protect the eyes and skin when working with concentrated acids.
X-Ray Fluorescence[edit]
X-ray fluorescence (XRF) is a relatively nondestructive and rapid method for detecting the occurrence and concentration of major and trace elements (Na through U) in bulk samples. Element concentration can be used to infer such depositional conditions as paleo-redox levels, terrigenous influence, and detrital or biogenic content through geochemical proxies[16][18][150][151][152][153][154][155][156][157][158][159][160][161][162][163][164][165][166]. Interpretation of depositional conditions of fine-grained sediments is always enhanced when geochemical proxies are integrated with physical, biological, and chemical observations from outcrops, cores, and thin sections.
Vertical profiles taken through appropriately spaced samples or with an XRF scanner are helpful supplementary information for sequence-stratigraphic analysis, especially using proxies that estimate such key factors as detrital or biogenic content, redox levels, and terrigenous influence. For more information on this technique, see, for example, Norrish and Chappell[167], Tertian and Claisse[168], Fairchild et al.[169], Rollinson[170], and Fitton[171].
XRF is well suited for bulk chemical analysis of major and minor elements (i.e. Al, Ca, Fe, K, Mg, Mn, Mo, Na, P, S, Si, Ti) and trace elements (>1 ppm; i.e. Ba, Ce, Cd, Co, Cr, Cu, Ga, La, Mo, Nb, Ni, Rb, Rh, Sc, Sr, U, V, Zn, Zr).
Sample Preparation for Bulk Analysis[edit]
- XRF is a bulk analysis, so sample size must be several times larger than the largest grain size or particle in the rock. For most mudstones, this means a representative sample of about 8 g (approximately 2 cm3 cube) for quantitative XRF. Some laboratories can use as little as 0.05 g for a “semiquantitative” XRF analysis.
- The sample is crushed to an average grain size of a few millimeters, and then a representative subsample is selected and powdered. A powdered aliquot is typically about 100 ml (∼250 mg).
The powdered sample can then be analyzed directly (appropriate for trace elements) or mixed with a chemical flux, melted to make a homogeneous glass, and analyzed (useful for samples with a wide range of elemental abundances, especially Fe).
- If sample availability is an issue, powdered mudstone samples that have been extracted for molecular geochemical analysis can be used for XRF analyses. Alternatively, if XRF was conducted on samples that were powdered-only, those samples can be used for other analytical techniques.
XRF Scanning Spectrometer[edit]
- Samples must have a relatively planar face (e.g., slabbed core). The main limitation is the length of sample that can be scanned at one time.
- Spatial resolution can be selected, typically from 0.1 to 10 mm between locations (width from 2 to 15 mm); an analysis takes from 1.5 to 30 s per location, with approximately 0.5 s between scans (depending on the machine used).
- Output is a profile with typically the same suite of major and trace elements as for single sample outputs (Na through U).
- See Fitton[171], Jansen et al.[172], Rothwell et al.[173], Banerjee[174], and Liu et al.[175] for details on applications.
Portable XRF Spectrometer[edit]
- The power of this approach is that it allows taking the instrument to the sample instead of the sample to the instrument. It is potentially useful for relatively rapid acquisition of vertical profiles in cores or outcrops.
- Two categories of portable XRF spectrometers, both of which use an x-ray tube source, are used:
- The first category consists of handheld, radar-gunlike units that are applied to a sample (“point and shoot”) to analyze a spot typically 3–10 mm in diameter.
- The second category consists of portable, boxlike units with a compartment into which a relatively small sample is placed. This type tends to be higher powered and more flexible in use.
- All portable units represent some trade-off of range and number of elements, flexibility, and accuracy for portability and speed. In general, they can detect more than 25 elements but are more reliable for heavier elements.
- Key factors in choosing an appropriate unit include a range of elements detected, excitation range, detector resolution and total count rate, ability to modify x-ray beam, and software and display options.
- Serious consideration must be given to evaluating whether the time in the field is best spent examining the rocks and collecting representative samples for later analyses instead of analyzing on the spot. Optimum use in the field requires at least one person dedicated to the instrument and a second person locating and preparing sample locations.
Paleo-Redox Proxies[edit]
It is not a simple matter to decipher paleo-redox conditions during the formation of organic-carbon-rich fine-grained rocks. Hence, a series of geochemical proxies are used to interpret paleo-redox conditions.
These proxies are ratios of a redox-sensitive element to an element that does not fluctuate with redox conditions: the degree of pyritization (DOP = Fepyritic/[Fepyritic + Feacid soluble]), V/Cr, V/(V+Ni), Ni/Co, U/Th, and Mo/Al[18][21][151][176][177][178][179][180][181][182][183][156][158][162][184][185][186][187][188][189][190][191][192][193][194][195][196].
Clastic Dilution Proxies[edit]
- Geochemical proxies commonly used for inferring the amount of clastic dilution include the Ti/Al, Si/Al, and K/(Fe + Mg) ratios[16][18][21][150][152][153][154][155][156][159][162].
- Detritus has been also predicted from the concentration of potassium using the following equation[197][198]:
Paleo-Productivity Proxies[edit]
Examples of geochemical proxies used to infer past rates of primary production include TOC, TOC/Al, and TOC/Ptot[16][18][21][154][155][156][159][162][199][200][201][202].
Tips and Traps[edit]
- Do not apply strictly or in isolation from other data (proxies can be misleading if sediment has been reworked, thus altering the original concentrations).
- Examine a variety of proxies; integrate with physical, biological, and chemical observations from outcrops, cores, and thin sections; and then make interpretations based on preponderance of evidence, not on any single proxy.
X-Ray Powder Diffraction[edit]
X-ray powder diffraction (XRD) is a relatively rapid and nonconsumptive method for detecting the occurrence and concentration of mineral species and phases in bulk samples. It is a basic tool for determining mudstone composition and especially useful in differentiating clay-mineral types that are difficult to determine optically. Its output should be used to infer depositional conditions only when used in conjunction with optical microscopy, electron microscopy, or both (because XRD by itself cannot differentiate among detrital, biogenic, or diagenetic origin of any mineral species). For more information on XRD, see Hardy and Tucker[203], Bish and Post[204], Mumme et al.[205], Moore and Reynolds[206], or other standard references.
Sample Selection and Analytical Considerations[edit]
- Typically, 4–8 g of a mudstone sample is ground finely (<10 μm) and homogenized, and a 10–30 mg aliquot selected. The sample is spread on a homogenous holder and air-dried.
- The sample is inserted into an x-ray diffractometer and scanned through a wide range of incidence angles.
- Each analysis takes 20 minutes or less.
Tips and Traps[edit]
- Because of the small sample size, it is, as always, essential to understand the geological context of the samples analyzed.
- Detection is limited to minerals constituting as low as 0.5% of the volume of a sample.
- Differentiating specific types and mixtures of clay minerals typically requires more advanced treatment and multiple analyses (e.g., ion saturation, glycolation, heating to various temperatures). The degree and amount of change in crystal structure, especially of swelling clays, provides supplementary information essential to identification.
- XRD analysis is quite straightforward; interpretation of XRD output, however, requires care and experience, especially for complex mixtures of clay minerals.
- Inferring depositional conditions requires optical microscopy or electron microscopy or both, as discussed in the Microscopy section.
Total Organic Carbon Content[edit]
Vertical and lateral profiles of total organic carbon (TOC) content have been used for rapid screening of the quality and distribution of mudstones as source, reservoirs, and seals of hydrocarbons (Table 4). The TOC content of a rock is the result of the nonlinear interaction of the rates of organic-matter production, destruction, and dilution[16][18][159][162][207][208][209][210][211][212]. High TOC contents record the optimized combinations of moderately high production, low destruction, and low dilution rates. Low TOC contents can be the result of low production, high dilution, or high destruction rates, as well as high thermal maturity. As referenced in the section on Well-Log Tools (and discussed in Sequence sets and composite sequences[213]), the vertical profile of TOC has been related to physiographic setting and sequence-stratigraphic units (i.e., parasequence, parasequence set, sequence, and sequence-set scale; see discussions in Creaney and Passey[214], Bohacs[10], and Bohacs et al.[16]).
TOC content can be measured through direct combustion, modified direct combustion, indirect (by difference), and pyrolysis plus combustion products. Each method has advantages and disadvantages; see Peters and Cassa[215] for a detailed discussion. We recommend the direct combustion method for most mudstones.
Sample Selection and Analytical Considerations[edit]
A few considerations regarding sample selection and analysis include the following:
- Sample size is typically approximately 250 mg; richer rocks require smaller samples. (See discussion in the previous sections about selecting representative samples for sequence-stratigraphic analysis.)
- TOC is expressed as the weight percent of organic carbon in a sedimentary rock.
- TOC analyses are relatively rapid and inexpensive.
Tips and Traps[edit]
- This measure is useful for the identification of mudstones enriched in organic matter as well as for generating vertical profiles of TOC content in mudstone successions.
- Samples for reliable analyses should be at least several centimeters in thickness, free of modern-day plant roots, and relatively unweathered (as indicated by the lack of oxidation of such reactive minerals as pyrite).
- The measured values of mature samples need to be corrected to estimate the original values at the time of deposition because as thermal maturity increases, TOC decreases.
Rock-Eval® Pyrolysis: Hydrogen and Oxygen Indices[edit]
Rock-Eval® pyrolysis is a rapid screening method for estimating the hydrogen and oxygen content of organic matter and provides insights into (1) the character of the original organic material (algal versus land plant), (2) the preservational conditions in the depositional environment[216], and (3) the quality and distribution of hydrocarbon sources and reservoirs (Table 4). Vertical profiles of hydrogen indices (HIs) have been related to physiographic setting and sequence-stratigraphic units and surfaces[10][217][218]. For more information on the Rock-Eval method and parameters, see Espitalié et al.[219][220], Lafargue et al.[221], Peters[222], and Tissot and Welte[223].
Sample Selection and Analytical Considerations[edit]
- Sample size can range from 100 to 500 mg, depending on the TOC content; richer rocks require smaller samples. (See discussion in the previous sections about selecting representative samples for sequence-stratigraphic analysis.)
- The Rock-Eval analysis is relatively rapid and inexpensive, making it quite useful for screening many samples and generating vertical profiles in mudstone successions.
- It requires the measurement of TOC content to calculate HI and oxygen index (OI).
Rock-Eval® Parameters[edit]
- Measurements include three peaks, S1, S2, and S3, as well as Tmax.
- ‘’Hydrogen Index (HI):’’ HI = [S2/TOC] x 100 (mg “hydrocarbons”/g organic carbon). It estimates the quantity of pyrolyzable organic compounds or “hydrocarbons”.
- ‘’Oxygen Index (OI):’’ OI = [S3/TOC] x 100 (mg CO2/g TOC). It is a function of the type and preservational state of the original organic matter. It tends to be higher in terrigenous organic matter and in oxidized aquatic organic matter. It is used together with HI to make inferences about the organic-matter type.
- ‘’Production Index (PI):’’ PI = S1/[S1+S2]. It estimates the amount of free hydrocarbons in the rock, which is a function of porosity and thermal maturity.
- ‘’Tmax>/sub>:’’ It is the temperature at which the maximum amount of S2 is generated. It is related to thermal maturity (as well as the organic-matter type).
Tips and Traps[edit]
- Insights into the original organic-matter type and depositional conditions are best provided by cross-plots such as S2 versus TOC and HI versus OI. See Peters and Cassa[215] and Peters et al.[216] for more information.
- Insights into sequence stratigraphy are best provided by vertical profiles of HI and TOC plotted together with well-log and paleontological data.
- Rock-Eval results are significantly affected by the level of thermal maturation: HI and OI decrease with increasing maturity.
- Rock-Eval results are also affected by outcrop weathering (decreases HI and increases OI) and by staining by migrated hydrocarbons or oil-based drilling muds (increases S2 and HI).
- Rock-Eval analysis is not useful for rocks with less than 0.5 wt.% TOC.
Visual Kerogen Description and Palynology[edit]
The definition of the term “kerogen” has evolved through time. See the discussions in Hunt[224], Durand[225], Tissot and Welte[223], Tyson[207], and Vandenbroucke and Largeau[226]. Here, we define kerogen as the sedimentary organic matter that is not soluble in common organic solvents. Following TOC and Rock-Eval analyses, selected samples may undergo visual kerogen description to determine the type of organic material present in a mudstone. Selected samples may also be analyzed for palynomorph (e.g., spores) and phytoclast identification.
Organic material includes aquatic algae, bacterial debris, land plant parts (wood, cuticle, etc.), charcoal, spores, pollen, and bitumen. Commonly, the structure of the original material is preserved, and a variety of macerals can be identified under the microscope. Both visual kerogen and palynology analyses provide direct insights into the origin of organic-material particles and depositional conditions (including redox levels, proximity to terrigenous provenance, and paleoclimate from land-plant spores and pollen), biostratigraphy, and maturity. Vertical trends of appropriately spaced samples add an almost invaluable dimension to sequence-stratigraphic analysis. Sequence-stratigraphic surfaces are commonly marked by distinct changes in organic matter across them (see discussions and examples in Parasequences, Sequence sets and composite sequences[213], and Parasequence sets and depositional sequences[143]). Assemblages of palynomorphs in lacustrine mudstones are helpful for discerning lake-basin type[227], which then provides specific hypotheses about the associated sequence-stratigraphic expression[210][228].
During these analyses, organic material is separated from crushed samples of whole rock using a variety of physical and chemical techniques in succession (including ultrasonic disaggregation, heating, oxidation, surfactants, flotation, and centrifugation). The resulting concentrated organic material is examined by optical (including reflected and UV light) or electron microscopy or both, and the occurrence, character, abundance, and taphonomy of organic material separated from whole-rock samples are described. Sample size is generally about 1–5 g (0.5–2 cm3) as a function of the organic-matter content of the whole rock (estimated by TOC). For more information, see Durand[225], Tyson[207], Taylor et al.[38], and Vandenbroucke and Largeau[226].
Sample Selection and Analytical Considerations[edit]
- Sample suites should span the full range of lithofacies present in an interval, with similar facies collected at multiple stratigraphic levels.
- One can sometimes make good use of small pieces that have fallen off into the core box if there is not too much vertical shifting.
- Outcrop samples with some weathering can still be quite useful, as the first step in many processing protocols is oxidation.
- The presence of solid bitumen in rocks with low permeability suggests that some oil generation has occurred locally.
Tips and Traps[edit]
- Identification of particular types of organic matter is quite challenging, especially in thermally mature samples. This can cause varying interpretations by different analysts as well as complicate your own. Terminology also varies widely, and some translation between different analysts is commonly needed.
- Cutting samples can be used, but they are prone to contamination from cavings and the presence of drilling additives (such as lignite and walnut shells).
Molecular Geochemistry[edit]
Molecular geochemistry has also been used to characterize the organic matter present in mudstones and to make inferences about past depositional environments. Biomarkers are molecular-compound fossils that are structurally related to their precursors in once-living organisms[216]. The character of a bio-marker is a function of the original organism as modified during deposition and later thermal maturation and biodegradation. Hence, biomarkers can provide insights into the age and composition of the original mix of organic matter and such depositional conditions as redox levels, salinity, and water column stratification[216]. Vertical profiles of the type and abundance of molecular markers are needed for sequence-stratigraphic analyses—these profiles must be specifically acquired from representative samples to reveal long-term secular trends[210][229][230]. Samples need to be taken from equivalent facies or locations with respect to significant surfaces (e.g., parasequence boundaries) to discern secular trends accurately. In lacustrine mudstones, small sample suites can be quite useful in determining the most likely lake-basin type[227], which then provides specific hypotheses about the sequence-stratigraphic expression in the strata[210][228]. For more information on analytical techniques and applications, see Philp and Gilbert[231], Philp[232], Schouten et al.[233], and Peters et al.[216].
Sample Selection and Analytical Considerations[edit]
- The size of rock samples is typically approximately 50 g (∼20 cm3 = 0.25 cm thick piece of a 4 in. slabbed core), although samples as small as 1–2 g can be analyzed successfully if they are sufficiently rich in organic matter.
- Screen the potential samples first for TOC content and Rock-Eval parameters. Molecular analyses are not recommended for samples with TOC <1 wt.%[234][235].
- The output of the molecular analysis represents the mixture of the many compounds of organic matter present in the tens of grams of a sample.
Tips and Traps[edit]
- Examine a variety of molecular markers; make interpretations based on preponderance of evidence and not on any single compound or ratio.
- Calculated molecular ratios may differ from laboratory to laboratory as a function of the instrument(s) and techniques used.
- Caution must be exercised in interpretation as biomarker character is the result of a long chain of transformations from photosynthesis to thermal maturation. For best results, use rock samples of low thermal maturity and, in the case of oil, of minimal biodegradation.
- Biomarkers represent only a tiny fraction of the organic matter, which itself is only a small part of the whole rock, so obtaining representative samples is essential, as is restraint in extrapolation (upscaling) of analytical results.
- All analyses are more meaningful when conducted on representative samples and interpreted within the geological context.
- Avoid contamination of samples: Enclose them in an aluminum foil (baked at ∼500°C) before wrapping or bagging in plastic. Do not label rock samples directly as marker pen ink is particularly problematic.
- Contamination with oil- and water-based drilling muds and mud additives may impact the results of molecular analyses.
Stable Isotopic Analysis[edit]
Isotopes are variants of a chemical element with different numbers of neutrons[236][237]. The two types of isotopes are unstable (radioactive) and stable (nonradioactive). Radioactive isotopes have provided the information needed for establishing the geological timescale. Stable isotopes have provided valuable insights into the origins of the component grains in mudstones, timing of cementation, and temperatures of precipitation[237][238][239]. In addition, stable isotopes have been used to link regional stratigraphy to events and settings characterized by distinct isotopic excursions from typical values present in adjacent sediments[240][241][242][243][244][245][246][247][248]. Stable isotopes of five elements, carbon (C), oxygen (O), hydrogen (H), sulfur (S), and nitrogen (N), have been used extensively for the study of mudstones. See discussion and references in Potter et al.[140] and Schieber[101]. A short introduction to C and O stable isotopes follows next.
The isotopic signature of a chemical element is expressed by the “delta” (δ) notation. The relative abundance of isotopes is recorded and reported as the ratio of the heavier-to-lighter isotopes in the sample compared to the same ratio in a chosen standard (e.g., the Vienna Peedee Belemnite for C and the Vienna Peedee Belemnite or the Vienna Standard Mean Ocean Water for O). Ratios of stable isotopes are expressed as parts per thousand (“per mil,” %). The varying stable-isotope ratios of elements such as C and O are present in mudstones in minerals such as calcite, quartz, and clay (see Potter et al.[140], appendix A.7, for examples of C and O isotopic variations in rocks and minerals). Isotopic values reflect varying contributions of detrital minerals, weathering products (e.g., clay minerals), cements, and organic materials[140][249], and are influenced by diagenetic processes and thermal maturity[250][251][252][253].
In sequence-stratigraphic studies, stable-isotope analyses can be used to refine the understanding of the origin of specific components, thereby aiding the interpretation of the environments of deposition[252][253][254]. Such data are particularly useful where they can show the changing balance of reservoirs that contributed solutes to specific reaction products. For instance, isotopic analyses of C in carbonate cements can be used to identify whether or not cements precipitated from (1) waters in equilibrium with the sea-water marine reservoir (δ13C typically on the order of 0%), (2) the anoxic pore waters associated with sulfate reduction (-25% δ13C), or (3) the anoxic conditions associated with methanogenesis (+15% δ13C)[255]. As part of these analyses, it is common to compare the δ13C data with data derived from δ18O (having made assumptions concerning starting isotopic composition of the pore waters), so that estimates of the temperature of cement precipitation can also be made.
Variations in the sources of organic carbon can also be tracked by isotopic analyses in mudstones as stable isotopic signatures of C vary depending upon the precise mechanisms of organic-carbon production and the subsequent degree of oxic degradations, and are thus very useful when relating variations in source-rock quality in sequence-stratigraphic context. For instance, because of the existence of different photosynthetic pathways and differences in the stable isotopic composition of C in the marine carbonate reservoir (0% δ13C) and CO2 in the atmosphere (-8% δ13C), marine phytoplankton typically exhibits stable isotopic values on the order of -10% to -31% δ13C, whereas land plants are typically in the range of -33% to -23% for C3 plants and -16% to -9% δ13C for C4 plants[256][257][258][259][260][261][262][263].
Sample Selection and Analytical Considerations[edit]
- Representative samples of each facies within the geological context are collected to enable robust interpretation and extrapolation.
- Generally, samples are combusted or pyrolyzed, and then the target gas species is purified by some combination of filters, traps, catalysts, and gas chromatography (GC). Following separation by GC, isotope ratios can be obtained using an isotope-ratio mass spectrometer.
- For inorganic carbon and oxygen of diagenetic cements, 50–100 mg of rock are used in the analysis. These samples are treated with acid to remove all organic matter before analysis.
- For organic carbon, 5–20 mg of rock extract is used in the analysis. Alternatively, 50–100 mg of whole rock is crushed and treated with acid to remove all carbonate carbon. Caution must be used, as the acid treatment can dissolve some labile carbon compounds (typically enriched in 13C) and thus change the isotopic value of the bulk carbon.
- For more information on the technique, see, for example, Faure[236], Hoefs[237], Coplen[264], Werner and Brand[265], and Lonstaffe[249].
Tips and Traps[edit]
- Samples should be as clean as possible to not introduce contaminants.
Reliable interpretation of stable isotopic data requires that the mudstones being analyzed have not undergone significant alteration since they accumulated (e.g., recrystallization or some other form of isotopic exchange), and the range of materials that are actually being sampled and analyzed is broadly known, particularly where sampling constraints mean that multiple grains or cement generations are being sampled.
- As for all analytical data, the most effective interpretations should always be made within a geological context.
Well-Log Tools[edit]
From bathtub experiments to modern nuclear physics, formation logging has been the backbone of oil and gas development, letting geologists, geophysicists and engineers see more and more of the subsurface. —Pike and Duey[266], p. 52
Well-log analysis is the most complete and least expensive method of characterizing mudstones down-hole. This method provides robust characterization of the stratigraphic distribution of potential source, reservoir, and seal rocks. It is particularly valuable where sample data are not available or for lake strata where thin-bedded source rocks are common. Few well-log types, however, directly measure the rock properties sought to evaluate source, reservoir, or seal potential—it is essential to understand the assumptions made, and caution must be used in interpretation.
The three main categories of well logs are electric, radioactive, and structural; most well logs were originally designed and mainly used for estimating lithology, porosity, rock strength, pressure, hydrocarbon presence and type, and structural characteristics (fractures, faults, folds, in situ stress). Table 5 summarizes the principles of well-logs commonly used for sequence-stratigraphic analysis and provides helpful tips and traps of log applications to mudstone successions. Many excellent textbooks provide more detail on well-logs and their interpretation[267][268][269][270][271]. A few general tips and traps to have in mind when using well logs are as follows:
- Direct measurement of rock properties from samples is always the most accurate information you can obtain and is invaluable for calibrating well-log estimates. Sample coverage, however, tends to be sparse and unevenly distributed; hence, calibrated well-logs are essential for evaluating the entire section under examination.
- Well-log estimates should be calibrated against values measured from appropriate samples whenever possible. Note, however, that the difference in sample volume analyzed versus that measured by a well-log tool (cm3 vs m3) makes caution essential in any calibration. See Passey et al.[272] for a discussion of this issue for organic-matter-rich mudstones.
- Many unusual lithologies can occur in mudstone successions, especially in those that accumulated in lake systems—and mudstones are not always identifiable by a high gamma-ray signature. Lacustrine rocks commonly pose special challenges to well-log analysis because of their thin beds and varied lithologies. Their well-log responses can differ significantly from marine rocks, especially with gamma-ray and resistivity tools[273][274][275].
- Well-log analyses of mudstones require special attention to the standard procedures for depth alignment in combined log techniques, tool calibration, and tool baseline. Pay particular attention to the caliper log, for some mudstones tend to wash out, whereas others drill “gunbarrel straight.”
For source evaluation, TOC depth profiles estimated from well-log data can be used to calculate TOC thickness, organic-matter volume, and the amount of hydrocarbon generated from a source rock over a particular area of a basin. The TOC profile also helps to interpret mudstone depositional environments and to correlate stratigraphic sequences[10][217][276]. Mudstone reservoir and seal character can be estimated through analogous analyses using combinations of various well-logs[277] (see Table 5); the vertical profiles generated also provide useful insights for constructing sequence-stratigraphic frameworks[10].
Seismic Tools[edit]
The unconformity-bounded sequences and their content comprise the most meaningful segments of the present stratigraphic record, without which valid interpretation of geologic history is patently impossible. —Wheeler[278], p. 600
This section is intended as an introduction and overview to this vast area of investigation. For more detail, we heartily recommend the reader to consult Payton[279], Sheriff[280], Abreu et al.[281], and Veeken and van Moerkerken[282].
Seismic data have been used over a large range from single wells to large basins such as across the Gulf of Mexico to provide continuous images of the subsurface to delineate stratal patterns and infer depositional history, environments of deposition, lithotypes, and fluid content[280][282].
Seismic stratigraphy is an essential component of sequence stratigraphy. Seismic stratigraphy involves both the (1) seismic-sequence analysis and (2) seismic-facies analysis. The seismic-sequence analysis subdivides the seismic section into geometrically defined intervals that are the seismic expression of depositional sequences—stratigraphic units of relatively conformable, genetically related strata bounded by unconformities and their correlative conformities. The seismic-facies analysis examines the configurations of reflections interpreted as strata within depositional sequences to determine the environmental setting and to estimate lithology[283]. Although there are numerous software tools for assisting these analyses, it is essential that the user understands the fundamentals to appropriately apply these tools.
Seismic Sequence Analysis[edit]
Seismic stratigraphy is the study of stratigraphy and depositional facies as interpreted from reflection seismic data. Seismic reflection terminations and configurations are interpreted as stratification patterns and are then used for recognition and correlation of depositional sequences, interpretation of depositional environment, and estimation of lithofacies[284]. It is basically a geological approach to interpreting geophysical data that aims to extract the maximum amount of information regarding paleogeomorphology, depositional environments, and facies through adding geological context and sedimentological insights.
Seismic reflections approximate bedding surfaces that record depositional profiles and therefore chronostratigraphic surfaces. This enables one to use the geometry of seismic reflection patterns to interpret
- chronostratigraphic correlations,
- genetically related depositional units,
- environments of deposition from depositional topography,
- paleobathymetry,
- topography and relief on unconformities,
- post-depositional structural deformation and syn-depositional thickness changes,
- burial history, and
- paleogeography and geological history.
Rock type cannot be determined directly from reflection patterns but can be estimated by the two-step process of interpreting environments of deposition and then using insights from models and observations of the depositional processes and facies.
Seismic-sequence analysis subdivides seismic sections into packages of concordant reflections (seismic sequences, interpreted as depositional sequences) that are separated by surfaces of discontinuity identified by systematic reflection terminations[284][285]. The two fundamental types of terminations are lapout and truncation. Lapout is the lateral termination of a stratum at its original depositional limit; truncation is the lateral termination of a stratum as a result of being cut off from its original depositional limit by subsequent erosion or deformation[284]. Reflection terminations interpreted as stratal terminations include onlap, downlap, toplap, and erosional truncation[284].
Seismic-Stratigraphy Procedure[edit]
The first step in seismic stratigraphy is to identify seismic terminations, and then use those terminations to recognize surfaces and delineate them. Next, key surfaces such as sequence boundaries and maximum flooding surfaces (maximum transgressive surfaces) are traced on the intersecting lines in a grid of seismic lines or propagated through a seismic-data volume until the boundaries have been correlated and tied within the entire dataset. This is intended to verify the regional extent of major discontinuity surfaces and to identify which other potentially significant surfaces are local in nature. Table 6 outlines the workflow for seismic stratigraphy. Table 7 details the criteria for identifying key stratigraphic surfaces.
Seismic Facies Analysis[edit]
Seismic-facies analysis is the description, mapping, and geologic interpretation of seismic-reflection parameters within a chronostratigraphic framework of sequence boundaries and downlap surfaces[284]. The interpreter delineates the external form, internal reflection characteristics, and 3-D associations of seismic-facies units—this enables interpretation of the units in terms of environmental setting, depositional processes, and estimates of lithotype. This interpretation is always done within a stratigraphic framework of depositional sequences to insure the analysis of genetically related strata. The genetically related stratal intervals are usually at the sequence or systems-tract scale but may range from sequence sets in very distal areas on 2-D regional lines to parasequences in sculpted subvolumes of 3-D data.
Basic seismic-facies information includes
- the relation of reflections to their upper and lower bounding surfaces (segments of onlap, downlap, toplap, truncation, and concordance; and direction of onlap and downlap), and
- the dominant types of reflection configuration between the upper and lower bounding surfaces (parallel, divergent, sigmoid, oblique, etc.).
In many instances, one aspect of the seismic facies proves more significant than others, and a separate map is prepared to portray that single aspect[284]. For example, dip direction of downlapping reflections is important in interpreting the sediment transport direction and possible provenance areas. In other cases, displaying some combination of characteristics, such as thickness and seismic amplitude, can be revealing.
Seismic-Facies Parameters: Internal Form[edit]
Seismic-facies units are mappable, 3-D seismic units composed of groups of reflections whose parameters differ from those of adjacent facies units[284]. These parameters include the configuration or geometry, continuity, amplitude, frequency, and interval velocity (Table 8). Each parameter provides considerable information on the geology of the subsurface (Table 8). Reflection configuration reveals gross stratification patterns from which depositional processes, erosion, and paleotopography can be interpreted. In addition, fluid contact reflections (flat spots) are commonly identifiable. Reflection continuity is closely associated with continuity of strata; continuous reflections suggest widespread, uniformly stratified deposits. Reflection amplitude contains information on the velocity and density contrasts of individual bedding interfaces and their spacing. It is used to predict lateral bedding changes and hydrocarbon occurrences. “Frequency” (reflection spacing), although a characteristic of the seismic pulse, is also related to such geologic factors as the spacing of reflectors or lateral changes in interval velocity (because of organic-matter or hydrocarbon content or lithofacies changes).
Grouping these seismic parameters into mappable seismic-facies units facilitates their interpretation in terms of depositional environment, sediment provenance, and geologic setting. Seismic-reflection configuration or geometry is the most obvious and directly analyzable seismic parameter. Stratal configuration or geometry is interpreted from seismic-reflection configuration and refers to the geometric patterns and relations of strata within a stratigraphic unit. These commonly indicate depositional setting and processes as well as later structural movement.
Seismic-Facies Mapping[edit]
Seismic-facies mapping involves analysis, on a qualitative or quantitative basis, of seismic character to infer areal trends in paleoenvironment and lithofacies. This section introduces a variety of techniques for mapping seismic-facies that can be used on the range of data from 2-D paper to 3-D volumes. The overall approach remains the same, but a particular technique is chosen based on dataset, resources, business stage, and objectives of study. Even with high-resolution 3-D datasets, it is generally useful to plot representative regional strike and dip lines on paper as a first step in defining the range of seismic facies and their rough distribution. Once the range of facies is defined, along with the dip and strike of the basin, then further interpretation and mapping is commonly conducted on a workstation.
Seismic-analysis computer programs can provide quantitative measures of frequency, continuity, and amplitude to support mapping. Seismic-amplitude mapping is particularly well developed in industry. Seismic-volume interpretation allows viewing of seismic-amplitude 3-D objects and their projected polygons in proper spatial and temporal relations.
As a quick reminder, some seismic parameters relate to lithofacies or the processes responsible for deposition. Other parameters relate to acoustic-impedance contrast, tuning, and the interaction of the seismic wave front with the strata, and thus, seismic frequency and resolution are factors in their patterns of occurrence. In the following discussion, stratal continuity is assumed to be detectable (i.e., continuity exceeds the Fresnel-zone width) for a given seismic frequency.
To extract the maximum geological information, one needs to map the smallest unit of genetically related strata, as a practical function of the objective of the interpretation project. For example, individual systems tract commonly have different seismic facies, and each needs its own map. Stacking all the systems tracts of one depositional sequence onto one map, by contrast, makes for a very complicated map that is difficult to interpret in terms of depositional environment or lithofacies[286].
External Form and Internal Geometry: A-B/C Mapping[edit]
Seismic-facies mapping was definitively explained by Ramsayer[285], based on 2-D seismic data. It is referred to as the “A-B/C” mapping approach, as observations are made upon the upper boundary (A), the lower boundary (B), and the internal reflection character (C). The A, B, and C categories of Ramsayer’s[285] seismic facies codes each included five types initially, thus providing 15 different variations for a given seismic interval of interest (Table 9). For example, a prograding seismic package with oblique clinoforms, toplap at its upper surface, and downlap at its base would be noted as Tp-Dn/Ob. Subsequent work and incorporation of Campbell’s[287] approach to stratal description expanded the range of descriptors for “C” (internal character) to span continuity, reflection shape, reflection inter-relations, and amplitude (Table 9, the lower part). We highly recommend using all these aspects of reflection character to describe the internal reflection configuration.
The A-B/C mapping is still necessary even with the most advanced and high-resolution 3-D datasets and visualization tools because seismic facies are most meaningful within their stratigraphic context—that is, when applied to genetically related strata (which is the whole point of sequence stratigraphy). Bounding surfaces provide important geological and stratigraphic information. Seismic facies are nonuniquely related to depositional environment and lithofacies (e.g., clinoform reflections can occur in deltaic sandstones, carbonate platforms, and mudstone-dominated shelves); hence, one needs more information from the stratigraphic context of the seismic facies—their relation to lower and upper bounding surfaces and position along the depositional profile.
Although the A-B/C technique was developed largely using 2-D seismic data, it can be used on 2-D and 3-D sections displayed on conventional industry workstations. The details vary according to the software tools used but generally involve defining a stratigraphic “pseudo-surface” for each depositional sequence or systems tract to represent its internal seismic facies and assigning a distinct time or depth value to represent each seismic facies. A 3-D dataset offers the advantages of allowing determination of the accurate direction of depositional dip and strike before selecting the orientation of the 2-D grid. Such datasets also allow the use of additional nonorthogonal lines to illustrate key stratigraphic relations and to aid interpolation. One can propagate and extend the initial grid of seismic facies interpretation using a variety of 3-D interpretation and visualization tools, some of which are discussed in the following section.
Seismic Facies: Emphasis on Amplitude Continuity[edit]
Since Ramsayer’s seminal paper in 1979[285], seismic facies techniques have evolved to include additional information on internal amplitude characteristics within individual systems tracts. Robust seismic facies information related to reflection amplitude, continuity, and frequency can be described in qualitative terms or quantified using various analysis techniques and software products[288]. This approach has been particularly well developed for deep-water paleoenvironments where amplitude commonly provides significant information on lithofacies and depositional environments (e.g., channel axis versus margin). This technique is most accurate where local well control is available to calibrate the amplitude-based seismic facies[289]. Calibrated observations of internal and external seismic character provide a means of interpreting depositional systems directly from seismic data in adjacent areas with little or no well control.
Seismic Facies by Trace Classification[edit]
Another innovation in seismic facies involves discrimination and classification of seismic wavelet trace shapes (using various computer programs). The approach must be used within an individual sequence or systems tract to extend what is essentially a one-dimensional seismic-facies analysis to a volume of genetically related strata.
Numerous other methods, both automated and semiautomated, for classifying the seismic data based on detailed geophysical parameters (frequency, wavelet duration, etc.) and on image analysis are available. Techniques using image analysis are particularly suitable for use in seismic facies mapping[288][290][291][292][293]. In one way, they represent just another way to describe and classify seismic facies and can be slotted into the general workflow at step 4 (Table 6). Remember that no matter how high-powered these techniques appear, they still must be applied to a genetically related volume of strata to provide the maximum amount of information on the depositional setting.
Techniques[edit]
The more technique you have, the less you have to worry about it. —Pablo Picasso[294]
This section details workflows for examining and interpreting mudstones across a broad range of scales, from μm to km, using the broad array of tools discussed previously in this chapter. We start with a workflow for sample-based analyses at the thin-section to outcrop scale. We then specify a workflow for sequence-stratigraphic analysis that summarizes key concepts for incorporating both sample and remote-sensing (well-log and seismic) data into a comprehensive stratigraphic framework. These workflows emphasize the appropriate and efficient use of the tools discussed in this chapter as well as the integration of observations of physical, chemical, and biogenic attributes of the rocks.
Integrated Workflow for Description of Mudstones in Outcrops, Cores, and Thin Sections[edit]
This section provides an introduction to the workflow we recommend for examining mudstones in outcrops, cores, and thin sections. Please see Lazar et al.[21] for more details on the specific steps that we take and the tools we recommend for describing these fine-grained rocks. Our approach is based on the fact that the appearance of mudstones can change significantly as a result of weathering in outcrops or cores (the relatively large content of reactive minerals such as clay and pyrite is very responsive to air and water and mudstones change readily). Describing these rocks in outcrops and cores is therefore a challenge, and, at first sight, one may argue that not much more than color variations can be observed. After preparing a fresh surface (Figure 1), however, detailed and systematic examination of mudstones reveals much about their texture, bedding, composition, vertical and lateral variation, and modes of accumulation. It also enables acquisition of appropriate and representative samples within their sedimentologic and stratigraphic context, and then further analyses under the microscope and in the laboratory.
The steps of the workflow we recommend for examining mudstones in outcrops, cores, and thin sections are:
- Make observations
- Establish stratigraphic context
- Step back, examine, and photograph the entire exposure, walking the section several times.
- Check core depths and core box order, clean the core, and photograph the entire core. Step back, examine, and walk the section several times with well logs in hand.
- Look for changes in texture, bedding, composition, thickness, continuity, and stacking; and presence of erosional surfaces. Tentatively identify stratigraphic packages.
- Make note of weathering characteristics and their vertical and lateral distribution.
- Start at the base of the section or core, identify, examine, and describe stratigraphic packages and surfaces as you proceed up-section. Integrate outcrop or core observations with thin-section observations. Observe and take pictures of stratigraphic features at all scales (laminae, laminasets, beds, bedsets, parasequences, parasequence sets, and sequences).
- Examine a fresh face of rocks from each potential stratigraphic package; sample each significant facies; mudstones tend to weather deeply and may require much digging to remove the weathered rock and to obtain fresh exposure and samples (Figure 1).
- Describe the mudstones: texture, bedding, and composition. Figure 1A–D in Mudstone nomenclature summarize the terms and definitions we recommend for texture, bedding, and composition. A practical, proxy method, the “scratch test,” we recommend to use to determine the dominant grain size at hand specimen scale is detailed in Table 10. Fine-tune outcrop and core estimations of texture, bedding, and composition by integrating observations under optical and electronic microscopes and analytical data obtained on samples taken in sedimentologic and stratigraphic context.
- Describe biogenic sedimentary structures and characterize the degree of bioturbation using a 0–5 scale (figure 1E of Mudstone nomenclature).
- Describe the type, size, diversity, abundance (figure 1F of Mudstone nomenclature), preservation, and taphonomy (figure 1G of Mudstone nomenclature) of body fossils. Similarly, describe trace fossils.
- Describe the type, size, composition, and abundance of diagenetic products (figures 1C, F of Mudstone nomenclature).
- Organize recurring, representative, and diagnostic facies attributes into facies associations.
- Identify and describe stratal packages and key surfaces (sequence boundaries and flooding surfaces; Figure 3; Tables 7, 11). Obtain a spectral gamma-ray profile to further characterize stratigraphic units (Figure 4).
- Record all information consistently in an appropriate format, designed for your particular setting or unit.
- Establish stratigraphic context
- Make interpretations
- Integrate outcrop, core, or thin-section observations with analytical, well-log, and seismic data as available. Make a hierarchical interpretation of stratal units (laminae, laminasets, beds, bedsets, parasequences, parasequence sets, sequences; significant stratal boundaries).
- Make interpretations of dominant sediment provenance, input mode, physical reworking, sediment accumulation rate, completeness of sedimentary record, bottom-water redox conditions, and environment of deposition[16][17][20][21] (e.g., Table 2 ; see also Laminasets, beds, and bedsets, Parasequences, and Parasequence sets and depositional sequences).
[[file:M126CH03-Figure3.jpeg|thumb|300px|Figure 3 Accommodation succession showing key stratigraphic surfaces, stacking patterns, and depositional sequence expression using definitions outlined in Tables 7 and 11[281] (after Neal and Abreu[295], and Abreu et al.[296]).]]
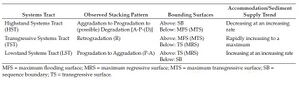
Introduction to Sequence Stratigraphy[edit]
The most perfect technique is that which is not noticed at all. —Pablo Casals[298]
The current concepts of sequence stratigraphy emerged from investigations of the relations among sedimentation, unconformities, and changes in base level[278][299][300][301][302][303][304][305][306][307][308] as well as from the development of seismic stratigraphy[283][309][310][311][312][313]. Concepts of sequence stratigraphy were put into practice by using the large-scale perspective of seismic cross sections and analysis of outcrop and well-log data within a chronostratigraphic framework[10][11][18][55][198][281][314][315][316][317][318][319][320].
Sequence stratigraphy was first applied to continental margin settings and has since expanded to address deep-water, fluvial, aeolian, mire, and lacustrine systems. The sequence-stratigraphic approach was explicitly extended to fine-grained sedimentary rocks beginning with the work on condensed sections (summarized in Loutit et al.[321]) and with fully integrated studies of source- and reservoir-prone mudstones from slope and basinal settings[9]Cite error: Closing </ref>
missing for <ref>
tag[322][323][324][325][274][326][327][328][329]. Each new environment sharpened our focus on the essentials and reinforced the validity of the basic approach. Although depositional processes and basin-filling mechanisms differ significantly, the basic approach of identifying key surfaces and recognizing repeated stratal geometries and stacking patterns works in all depositional settings. This approach is particularly essential in understanding the occurrence, character, and distribution of mudstones, and therefore, it is quite essential to locate and extract natural resources effectively and efficiently.
Key Concepts of Sequence Stratigraphy[edit]
Sequence stratigraphy is the study of rocks within a framework in which the vertical succession of rocks is subdivided into genetically related 3-D units that have characteristic stacking patterns and physical, biogenic, and chemical properties, and are bounded by surfaces, including unconformities and their correlative conformities[9][10][281][283][309][310][311][312][313][330][331][332]. Sequence stratigraphy has five major components[9][10][281]:
- ‘’Method’’ that recognizes a hierarchy of various types of stratal surfaces, rock packages, and stacking patterns.
- ‘’Observations’’ of physical, biological, and chemical aspects of surfaces and rocks within a 3-D framework.
- ‘’Models’’ that summarize and generalize detailed observations.
- ‘’Mechanisms’’ that seek to explain the origin of stratal patterns and facies distributions in terms of small-to-large-scale processes and to provide predictive capabilities.
- ‘’Prediction and Testing’’ that uses further observations to test predictive capabilities, refine models, and enhance the understanding of key mechanisms.
Sequence stratigraphy allows the construction of a comprehensive stratigraphic framework based on a single criterion—the physical relations of the strata themselves—that reveals genetically related rocks. Additionally, a comprehensive sequence-stratigraphic framework is ideal for integrating all types of data, from reflection seismic, through well-log, core, outcrop, thin section, to organic and inorganic geochemistry. This integrated response allows recognition, correlation, and prediction of sequence-stratigraphic units and surfaces in all these datasets across all scales.
The main building blocks of the stratigraphic record are depositional sequences that contain parasequences composed of beds and bedsets. All of these stratal building blocks, although discontinuous at some scale, comprise relatively conformable successions of smaller scale units bounded by surfaces of erosion, nondeposition, or correlative conformity.
Sequence stratigraphy is an informal method that combines various chronostratigraphic approaches that have evolved over the past 400 years. It organizes and systematically applies stratigraphic insights from Steno’s[333] principles of superposition, lateral continuity, and original horizontality, through Smith’s[334] and Lyell’s[335][336] rock systems, Walther’s[337] law of facies succession, Chamberlain’s[338][339] and Sloss’s[305][306] unconformity-bounded cratonic sequences, and Van Siclen’s[340] depositional topography, to the Exxon group’s lamina, laminaset, bed, and bedset, and pattern correlation[287], seismic stratigraphy[310][311][312], parasequences[17]Cite error: Closing </ref>
missing for <ref>
tag, to today’s fully integrated sequence stratigraphy[16][18][327][341].
Throughout the following discussion it is essential to remember that the rock record is fundamentally discontinuous, with breaks in sediment accumulation at all scales, from kilometers to submillimeters. Hence, the terms “isochronous” or “diachronous” and “conformable” or “unconformable” are to be understood in relative terms that are a function of the resolution of data being used to make the interpretation. Practically, all surfaces result from a change in the sediment accumulation rate at some level of significance—a supercritical decrease or increase in the rate of sediment supply relative to the accommodation rate that produces a change in system behavior. (Level of significance refers to the spatial (and inferred temporal) distribution of the surface and the rocks it bounds—how much area it covers and how much time it records.) This change in system behavior results in erosion, nondeposition, or different rock characteristics (texture, bedding, or composition). Our observations indicate that most surfaces record a pause in sediment accumulation.
Stratal Units and Surfaces[edit]
This section presents an overview of all scales and components of the stratal hierarchy used in sequence stratigraphy. They are all elaborated in the following chapters. Stratal Units—Sequence-stratigraphic stratal units are defined using geometric criteria, with the supporting evidence of other physical, biogenic, and chemical attributes. Although thickness, areal extent, and time for formation are neither essential attributes nor part of the definition of sequence-stratigraphic units, these units do tend to have characteristic spatial and temporal scales as well as common modes of formation. Note that characteristic thicknesses tend to be a function of grain size and are typically thinner in mudstones. Characteristic timescales tend to be strongly related to depositional setting and basin size, with relatively short intervals in small lacustrine basins and relatively long intervals in large marine basins (according to the response time of the basin, which scales to the second power of its characteristic length scale; see Paola et al.[342]).
The ‘’depositional sequence’’ is the fundamental unit of sequence stratigraphy; it is a relatively conformable succession of strata bounded at base and top by laterally extensive (regional scale) unconformities and their correlative conformities[281][295][330] (Figure 3). Depositional sequences are meters to hundreds of meters thick and extend over many thousands of square kilometers. They are inferred to represent multiple episodes of shoreline progradation with significant shifts in coastal onlap and base level over tens to thousands of millennia. A complete depositional sequence can be subdivided into ‘’systems tracts’’ defined by their position within the sequence and by the stacking patterns of the ‘’parasequence sets’’ within each systems tract. Parasequence sets are bounded by ‘’parasequence set boundaries’’ that are ‘’flooding surfaces’’ and their equivalents. Systems tracts include lowstand, trans-gressive, and highstand (see Table 11).
A ‘’parasequence’’, the main building block of the depositional sequence, is a relatively conformable succession of beds or bedsets bounded below and above by parasequence boundaries (surfaces that record a pause in sediment accumulation, formed by nondeposition, local erosion, or very slow sedimentation and include flooding, abandonment, or reactivation surfaces and their correlative surfaces; after Van Wagoner et al.[316][332]; Bohacs[10], Bohacs et al.[17]). Parasequences range from tens of centimeters to tens of meters in thickness and extend over significant parts of a basin, on the order of hundreds to thousands of square kilometers. In shelf or lacustrine settings, they typically represent one episode of shoreline or mudbelt progradation—the dominant depositional “motif” or building block. Equivalent-scale units in other settings include fan “lobes” in submarine-fan settings and channel-belt sets in fluvial or submarine-slope settings. Parasequences are interpreted to form in centuries to millennia.
A ‘’bed’’ is a relatively conformable succession of genetically related laminae or laminasets bounded at base and top by bedding surfaces—surfaces of erosion, nondeposition, or correlative conformity[287].
A ‘’lamina’’ is the smallest megascopic layer in a sedimentary succession without internal layers[287] (typically ≥0.1 mm or so). See Mudstone nomenclature, and Laminasets, beds, and bedsets for detailed discussions of laminae, beds, and parasequences.
Stratal Surfaces[edit]
The two distinct types of widespread and mappable surfaces are parasequence boundaries and sequence boundaries[9][10][283][309][310][313][331][332][343] (Figure 3). Identification of these surfaces in a stratal succession relies on both their local character and their lateral extent[9][10][17][18][20][21][281][316][343].
A ‘’parasequence boundary’’ (‘’flooding surface’’ and correlative surfaces) records a supercritical increase in accommodation relative to sediment supply that significantly changes system behavior[10][343]. Commonly, strata above a parasequence boundary are deposited in deeper water, and less energetic and more distal environments, whereas strata below a flooding surface are deposited in shallower water, and more energetic and proximal environments[10][17][20][21][343]. Parasequence boundaries are marked by a sharp decrease in coarse sediment supply, increased and laterally extensive accumulation of pelagic and authigenic components (e.g., organic matter, remains of plankton and nekton, volcanic ash, dropstones; cements, nodules, and concretions), early lithification or cementation, and increased continuity of laminae, beds, and bedsets[9][10][17][55]Cite error: Closing </ref>
missing for <ref>
tag.
‘’Sequence boundaries’’ are the laterally extensive (regional scale) unconformities and correlative conformities that bound a depositional sequence[330]; they are fundamentally different from flooding surfaces. Sequence boundaries (in contrast to flooding surfaces) record a supercritical decrease in accommodation relative to sediment supply, commonly accompanied by an increase in depositional energy or a significant change in sediment supply (e.g., erosional bypass in marine environments), over hundreds to thousands of square kilometers[10][18][343][344][345]. They are easiest to recognize in medial reaches of the shelf. Common attributes of sequence boundaries are summarized in Table 7 and discussed further in Parasequence sets and depositional sequences. Sequence boundaries are surfaces across which there is a basinward shift in coastal onlap, marked by laterally extensive erosional truncation of underlying strata (with evidence of exposure and presence of reworked clastics in lag deposits) and toplap below and onlap and downlap above[10][11][18][198][330][343][344]. It occurs below the abrupt basinward shift in shoreline position at the base of a depositional sequence. It is placed at the surface beneath the first increase in accommodation above progradationally or degradationally stacked parasequences, at the break in shoreline sandstone trajectory (Figure 3).
Constructing and Testing a Sequence-Stratigraphic Framework for Mudstones[edit]
As discussed in Bohacs and Lazar[346], ‘method, observations, models, mechanisms’, and ‘prediction and testing’ are the five major components of sequence stratigraphy[9][10][281]. An optimal workflow for establishing a sequence-stratigraphic framework follows this fivefold organization and includes the following[9][10]:
- Establishing geological context and geophysical background of the region under investigation
- Identifying large-scale stratal units by locating terminations and interpreting surfaces
- Tying surfaces and stratal units around a grid
- Making maps of terminations, stratal units, and their internal geometry
- Interpreting depositional environments and estimating facies character from those maps and sections
- Summarizing and generalizing observations and first-order interpretations into a model for the 3-D distribution of surfaces, strata, and rock properties
- Inferring the mechanisms that led to the formation of the surfaces and strata, nesting small-scale processes of grain formation, erosion, transport, and deposition within larger scale processes of climate, tectonics, and biological evolution and their forcing functions
Details of this workflow are given in Table 12 and elaborated throughout the following chapters.
Now, we can proceed to apply these tools and techniques at successively larger scales, starting with the smallest scales, laminae, laminasets, beds, and bedsets.
Conclusions[edit]
Mudstone vertical and lateral variability at millimeter to kilometer scales can be detected by applying a range of physical, petrophysical, chemical, and paleontological methods to characterize and interpret the rocks within an integrated sequence-stratigraphic approach. This chapter introduced key tools and techniques that provide data about texture, bedding, composition, and grain origin. Such data enable characterization of mudstone strata at lamina to sequence-set scales. The application of such tools and techniques to decipher depositional conditions and construct sequence-stratigraphic frameworks was specifically addressed. Outlines of our approach to making detailed and systematic observations of key attributes of mudstones in outcrops, cores, and thin sections as well as an introduction to key sequence-stratigraphic concepts that we find useful for studying mudstones were included for quick reference. Our approach is elaborated and illustrated in all of the following chapters.
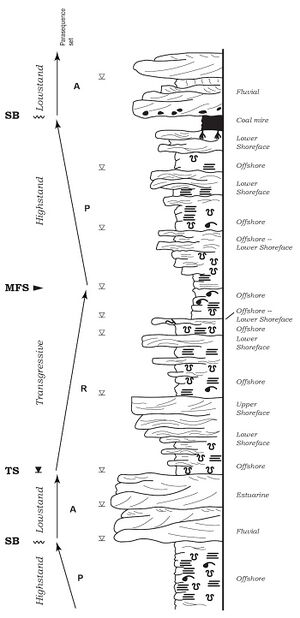
See also[edit]
Case studies ch 9-15
Further Reading[edit]
- Embry, A. F., and O. Catuneanu, 2002, Practical sequence stratigraphy: Concepts and applications: Canadian Society of Petroleum Geologists Short Course Notes, p. 167.
- Herron, M., and S. L. Herron, 1990, Geological applications of geochemical well logging, in A. Hurst, M. A., Lovell, and A. C., Morton, eds., Geological applications of wireline logs: Geological Society (London) Special Publication 48, p. 165–175.
- Herron, S. L., and M. M. Herron, 1996, Quantitative lithology: An application for open and cased hole spectroscopy: Society of Petrophysicists and Well Log Analysts 37th Annual Logging Symposium, p. 1–14.
- Meyer, B. L., and M. H. Nederlof, 1982, Identification of source rocks on wireline logs by density/resistivity and sonic transit time/resistivity cross-plots: AAPG Bulletin, v. 68, p. 121–129.
- Myers, K. J., 1987, Onshore outcrop gamma-ray spectrometry as a tool in sedimentological studies, Ph.D. thesis, University of London, London, 180 p.
- Myers, K. J., and P. B. Wignall, 1987, Understanding Jurassic organic-rich mudrocks: New concepts using gamma ray spectrometry and paleoecology: Examples from the Kimmeridge Clay of Dorset and the Jet Rock of Yorkshire, in J. K. Leggett, and G. G. Zuffa, eds., Marine clastic environments: Concepts and case studies: London, Graham and Trotman, p. 175–192.
- Passey, Q. R., K. M. Bohacs, W. L. Esch, R. Klimentides, and S. Sinha, 2010, From oil-prone source rock to gas-producing shale reservoir—geological and petrophysical characterization of unconventional shale gas reservoirs: International Oil and Gas Conference in China, Beijing, China, 8-10 June 2010: SPE 131350-MS, 29 p.
- Popielski, A. C., Z. Heidari, and C. Torres-Verdín, 2012, Rock classification from conventional well logs in hydrocarbon bearing shale: SPE Annual Technical Conference and Exhibition, San Antonio, Texas, October 8–10: SPE 159255-PP.
- Radtke, R. J., M. Lorente, B. Adolph, M. Berheide, S. Fricke, J. Grau, S. Herron, et al., 2012, A new capture and inelastic spectroscopy tool take geochemical logging to the next level: Society of Petrophysicists and Well Log Analysts, 53rd Annual Logging Symposium, Cartagena, Columbia, June 16–20, 2012, p. 1–16.
- Schieber, J., 1986, The possible role of benthic microbial mats during the formation of carbonaceous shales in shallow mid-Proterozoic basins: Sedimentology, v. 33, p. 521–536.
- Schlumberger, C., M. Schlumberger, and H. G. Doll, 1932, Electrical coring: A method of determining bottom-hole data by electrical measurements: American Institute of Mining Engineering Technical Paper 462, SPE-934237-G.
- Vinther, R., and K. Mosegaard, 1996, 3D seismic texture classification: SPE 35482, p. 57–63.
- Yau, Y. C., D. R. Peacor, and S. D. McDowell, 1987, Smectite-to-illite reactions in Salton Sea shales: A transmission and analytical electron microscopy study: Journal of Sedimentary Petrology, v. 57, p. 335–342.
- Zelt, F. B., 1985, Natural gamma-ray spectrometry, lithofacies, and depositional environments of selected upper Cretaceous marine mudrocks, Western United States, including Tropic Shale and Tununk member of Mancos Shale, Ph.D. dissertation, Princeton University, Princeton, New Jersey, 301 p.
- Zhu, Y., E. Liu, A. Martinez, M. A. Payne, and C. E. Harris, 2011, Understanding geophysical responses of shale-gas plays: The Leading Edge, March 2011, p. 332–338.
- Zhu, Y., S. Xu, M. Payne, A. Martinez, E. Liu, C. Harris, and K. Bandyopadhyay, 2012, Improved rock physics model for shale gas: SEG Conference, Las Vegas, Nevada, November 5–9, 2012, SEG-2012-0927, 5p.
References[edit]
- ↑ 1.0 1.1 1.2 Potter, P. E., J. B. Maynard, and W. A. Pryor, 1980, Sedimentology of shale, Study guide and reference source: Berlin, Springer-Verlag, 303 p.
- ↑ Sorby, H. C., 1908, On the application of quantitative methods to the study of the structure and history of rocks: Quarterly Journal of the Geological Society, v. 64, p. 171–233.
- ↑ Picard, D. M., 1971, Classification of fine-grained sedimentary rocks: Journal of Sedimentary Petrology, v. 41, p. 179–195.
- ↑ Stow, D. A., 1981, Fine-grained sediments: Terminology: Quarterly Journal of Engineering Geology and Hydrology, v. 14, p. 243–244.
- ↑ Blatt, H., 1982, Sedimentary petrology: New York, W. H. Freeman, 564 p.
- ↑ Klemme, H. D., and G. F. Ulmishek, 1991, Effective petroleum source rocks of the world: Stratigraphic distribution and controlling depositional factors: AAPG Bulletin, v. 75, p. 1809–1851.
- ↑ Curtis, J. B., 2002, Fractured shale-gas systems: AAPG Bulletin, v. 86, p. 1921–1938.
- ↑ Curtis, J. B., 2009, The contribution of shale gas to future US production: A view of the resource base: AAPG Search and Discovery Article #110082.
- ↑ 9.0 9.1 9.2 9.3 9.4 9.5 9.6 9.7 9.8 9.9 Bohacs, K. M., and J. R. Schwalbach, 1992, Sequence stratigraphy of fine-grained rocks with special reference to the Monterey Formation, ‘’in’’ J. R. Schwalbach, and K. M. Bohacs, eds., Sequence stratigraphy in fine-grained rocks: Examples from the Monterey Formation: SEPM Field Trip Guidebook—the Pacific Section 70, p. 7–20.
- ↑ 10.00 10.01 10.02 10.03 10.04 10.05 10.06 10.07 10.08 10.09 10.10 10.11 10.12 10.13 10.14 10.15 10.16 10.17 Bohacs, K. M., 1998, Contrasting expressions of depositional sequences in mudrocks from marine to nonmarine environs, ‘’in’’ J. Schieber, W. Zimmerle, and P. Sethi, eds., Shales and mudstones I.: Stuttgart, Germany, E. Schweizerbart’sche Verlagsbuchhandlung (Nagele u. Obermiller), p. 33–78.
- ↑ 11.0 11.1 11.2 11.3 Schieber, J., 1998a, Developing a sequence stratigraphic framework for the Late Devonian Chattanooga Shale of the southeastern USA: Relevance for the Bakken Shale, ‘’in’’ J. E. Christopher, C. F. Gilboy, D. F. Paterson, and S. L. Bend, eds., 8th International Williston Basin Symposium, October 19–21, 1998, Regina, Saskatchewan: Saskatchewan Geological Society Special Publication 13, p. 58–68.
- ↑ 12.0 12.1 12.2 Schieber, J., 1998b, Sedimentary features indicating erosion, condensation, and hiatuses in the Chattanooga Shale of Central Tennessee: Relevance for sedimentary and stratigraphic evolution, ‘’in’’ J. Schieber, W. Zimmerle, and P. Sethi, eds., Shales and mudstones, Volume I: Stuttgart, E. Schweizerbart’sche Verlagsbuchhandlung (Nagele u. Obermiller), p. 187–215.
- ↑ 13.0 13.1 13.2 13.3 13.4 13.5 13.6 Schieber, J., 1999, Distribution and deposition of mudstone facies in the Upper Devonian Sonyea Group of New York: Journal of Sedimentary Research, v. 69, p. 909–925.
- ↑ 14.0 14.1 14.2 Macquaker, J. H. S., R. L. Gawthorpe, K. G. Taylor, and M. J. Oates, 1998, Heterogeneity, stacking patterns and sequence stratigraphic interpretation in distal mudstone successions: Examples from the Kimmeridge Clay Formation, U.K., ‘’in’’ J. Schieber, W. Zimmerle, and P. Sethi, eds., Shales and mudstones, Volume I: Stuttgart, Germany, E. Schweizerbart’sche Verlagsbuchhandlung (Nagele u. Obermiller), p. 163–186.
- ↑ Macquaker, J. H. S., K. G. Taylor, and R. L. Gawthorpe, 2007, High-resolution facies analyses of mudstones: Implications for paleoenvironmental and sequence stratigraphic interpretations of offshore ancient mud-dominated successions: Journal of Sedimentary Research, v. 77, p. 324–339.
- ↑ 16.0 16.1 16.2 16.3 16.4 16.5 16.6 16.7 Bohacs, K. M., G. J. Grabowski Jr., A. R. Carroll, P. J. Mankiewicz, K. J. Miskell-Gerhardt, J. R. Schwalbach, M. B. Wegner, and J. A. Simo, 2005, Production, destruction, and dilution—the many paths to source-rock development, ‘’in’’ N. B. Harris, ed., The deposition of organic-carbon-rich sediments: Models, mechanisms, and consequences: SEPM Special Publication 82, p. 61–101.
- ↑ 17.0 17.1 17.2 17.3 17.4 17.5 17.6 17.7 17.8 Bohacs, K. M., O. R. Lazar, and T. M. Demko, 2014, Parasequence types in shelfal mudstone strata—quantitative observations, lithofacies stacking patterns, and a conceptual link to modern depositional regimes: Geology, v. 42, p. 131–134.
- ↑ 18.00 18.01 18.02 18.03 18.04 18.05 18.06 18.07 18.08 18.09 18.10 18.11 18.12 18.13 Lazar, O. R., 2007, Redefinition of the New Albany Shale of the Illinois basin: An integrated, stratigraphic, sedimentologic, and geochemical study, Ph.D. thesis, Indiana University, Bloomington, Indiana, 336 p.
- ↑ Lazar, O. R., K. M. Bohacs, J. H. S. Macquaker, and J. Schieber, 2010, Fine-grained rocks in outcrops: Classification and description guidelines, in J. Schieber, O. R. Lazar, and K. M. Bohacs, eds., Sedimentology and stratigraphy of shales: Expressions and correlation of depositional sequences in the Devonian of Tennessee, Kentucky, and Indiana: AAPG Field Guide for SEPM Field Trip 10, p. 3–14.
- ↑ 20.00 20.01 20.02 20.03 20.04 20.05 20.06 20.07 20.08 20.09 20.10 20.11 20.12 Lazar, O. R., K. M. Bohacs, J. H. S. Macquaker, J. Schieber, and T. M. Demko, 2015a, Capturing key attributes of fine-grained sedimentary rocks in outcrops, cores, and thin sections: Nomenclature and description guidelines: Journal of Sedimentary Research, v. 85, p. 230–246.
- ↑ 21.00 21.01 21.02 21.03 21.04 21.05 21.06 21.07 21.08 21.09 21.10 21.11 21.12 21.13 21.14 21.15 21.16 21.17 21.18 21.19 21.20 21.21 21.22 21.23 21.24 21.25 21.26 Lazar, O. R., K. M. Bohacs, J. Schieber, J. H. S. Macquaker, and T. M. Demko, 2015b, Mudstone primer: Lithofacies variations, diagnostic criteria, and sedimentologic/stratigraphic implications at lamina to bedset scale: SEPM Concepts in Sedimentology and Paleontology 12, 200 p.
- ↑ Berra, Y., 2010, The Yogi book: New York, Workman, 175 p.
- ↑ Lazar, O. R., K. M. Bohacs, J. Schieber, J. H. S. Macquaker, and T. M. Demko, 2022a, Mudstone nomenclature, in K. M. Bohacs and R. Lazar, eds., Sequence stratigraphy: Applications to fine-grained rocks: AAPG Memoir 126, p. 21–34.
- ↑ Ekdale, A. A., R. G. Bromley, and S. G. Pemberton, 1984, Ichnology: Trace fossils in sedimentology and stratigraphy: SEPM Short Course Notes, v. 15, p. 317.
- ↑ 25.0 25.1 Miller, J., 1988, Microscopical techniques: I. Slices, slides, stains and peels, in M. E. Tucker, ed., Techniques in sedimentology: Oxford, U.K., Blackwell Scientific Publications, p. 86–107.
- ↑ Scholle, P. A., and D. Ulmer-Scholle, 2003, A color guide to the petrography of carbonate rocks: Grains, textures, porosity, diagenesis: AAPG Memoir 77, 474 p.
- ↑ 27.0 27.1 27.2 Flügel, E., 2010, Microfacies of carbonate rocks: Analysis, interpretation and application, 2nd ed.: Berlin, Springer-Verlag, 984 p.
- ↑ Frey, R. W., 1970, The Lebenspurren of some common marine invertebrates near Beaufort, North Carolina. 2, Anemone burrow: Journal of Paleontology, v. 44, p. 308–311.
- ↑ Hamblin, W. K., 1962, Staining and etching techniques for studying obscure structures in clastic rocks: Journal of Sedimentary Petrology, v. 32, p. 201–210.
- ↑ Farrow, G. E., 1966, Bathymetric zonation of Jurassic trace fossils: Palaeogeography, Palaeoclimate, Palaeoecology, v. 2, p. 103–151.
- ↑ West, I. M., 1965, A new method of displaying microstructures in porous limestone: Journal of Sedimentary Petrology, v. 35, p. 250–251.
- ↑ Risk, M. J., and R. B. Szczuczko, 1977, A Method for Staining Trace Fossils: Journal of Sedimentary Petrology, v. 47, no. 2, p. 855–859.
- ↑ 33.0 33.1 33.2 33.3 Friedman, G. M., 1959, Identification of carbonate minerals by staining methods: Journal of Sedimentary Petrography, v. 29, p. 87–97.
- ↑ 34.0 34.1 Evamy, B. D., 1963, The application of a chemical staining technique to a study of dedolomitisation: Sedimentology, v. 2, no. 2, p. 164–170. doi:10.1111/j.1365-3091.1963.tb01210.x.
- ↑ Yanguas, J. E., and J. J. Dravis, 1985, Blue fluorescent dye technique for recognition of microporosity in sedimentary rocks: Journal of Sedimentary Petrology, v. 55, no. 4, p. 600–602.
- ↑ Bushinsky, G. I., 1947, Textures and structures of Cretaceous chalk and flints (Russian with English summary): Bulletin of Society of Naturalists, Moscow, New Series 52 (Geology), v. 22, p. 37–43.
- ↑ Bromley, R. G., 1981, Enhancement of visibility of structures in marly chalk: Modification of the Bushinsky oil technique: Bulletin of the Geological Society of Denmark, v. 29, p. 111–118.
- ↑ 38.0 38.1 Taylor, G. H., M. Teichmuller, A. Davis, C. F. K. Diessel, R. Littke, and R. Robert, 1998, Organic petrology: Berlin, Stuttgart, Gebruder Borntraeger, 704 p.
- ↑ 39.0 39.1 39.2 Lobza, V., and J. Schieber, 1999, Biogenic sedimentary structures produced by worms in soupy, soft muds: Observations from the Chattanooga Shale (Upper Devonian) and experiments: Journal of Sedimentary Research, v. 69, p. 1041–1049.
- ↑ 40.0 40.1 40.2 40.3 Schieber, J., 2003, Simple gifts and buried treasures—implications of finding bioturbation and erosion surfaces in black shales: The Sedimentary Record, v. 1, p. 4–8.
- ↑ Hooke, R., 1667, Micrographia: or, some physiological descriptions of minute bodies made by magnifying glasses. with observations and inquiries thereupon: Sagwan Press 2015 edition, J. Martyn, and J. Allestry, J., eds., 320 p.
- ↑ Catt, J. A., and P. C. Robinson, 1961, The preparation of thin sections of clays: Geological Magazine, v. 98, p. 511–514.
- ↑ Altemüller, H.-J., 1974, Mikroskopie der Böden mit Hilfe von Dünnschliffen, in H. Freund, ed., Handbuch der Mikropskopie in der Technik, 4, part 2: Frankfurt, Germany, Umschau Verlag, p. 309–367.
- ↑ Murphy, C. P., 1986, Thin section preparation of soils and sediments: Berkhamsted, U.K., AB Academic Publishing, 149 p.
- ↑ Lindholm, R. C., 1987, A practical approach to sedimentology: London, Allen and Unwin, 276 p.
- ↑ 46.0 46.1 O’Brien, N. R., 1981, SEM study of shale fabric—A review: Scanning Electron Microscopy, v. 1, p. 569–575.
- ↑ Zimmerle, W., 1982, Sedimentologische Dünnschliff-Analyse der dunklen Tonsteine von Ober-Apt und Unter-Alb (Niedersächsisches Becken), ‘’in’’ E. Kemper, ed., Das Späte Apt und frühe Alb Nordwestdeutschlands, Versuch der umfassenden Analyse einer Schichtenfolge. Geologisches Jahrbuch. Reihe A: Allgemeine und Regionale Geologie BR Deutschland und Nachbargebiete, Tektonik, Stratigraphic, Paläontologie, 65, p. 63–109.
- ↑ Zimmerle, W., 1994, On the lithology of the Rupelian Boom Clay in northern Belgium, a volcaniclastic deposit: Bulletin Societé Belge de Géologie, v. 102, p. 91–103.
- ↑ 49.0 49.1 49.2 Schieber, J., 1989, Facies and origin of shales from the Mid-Proterozoic Newland Formation, Belt basin, Montana, USA: Sedimentology, v. 36, p. 203–219.
- ↑ 50.0 50.1 Schieber, J., 2002, Sedimentary pyrite: A window into the microbial past: Geology, v. 30, p. 531–534.
- ↑ 51.0 51.1 Schieber, J., 2009, Discovery of agglutinated benthic foraminifera in Devonian black shales and their relevance for the redox state of ancient seas: Paleogeography, Paleoclimatology, Paleoecology, v. 271, p. 292–300.
- ↑ 52.0 52.1 52.2 Schieber, J., 2011, Shale microfabrics and pore development—An overview with emphasis on the importance of depositional processes, in D. A. Leckie, and J. E. Barclay, eds., Gas shale of the Horn River basin: Calgary, Canada, Canadian Society of Petroleum Geologists, p. 115–119.
- ↑ 53.0 53.1 O’Brien, N. R., and R. M. Slatt, 1990, Argillaceous rock atlas: New York, Springer-Verlag, 141 p.
- ↑ 54.0 54.1 54.2 Macquaker, J. H. S., and R. L. Gawthorpe, 1993, Mudstone lithofacies in the Kimmeridge Clay Formation, Wessex Basin, southern England: Implications for the origin and controls of the distribution of mudstones: Journal of Sedimentary Petrography, v. 63, p. 1129–1143.
- ↑ 55.0 55.1 55.2 55.3 55.4 55.5 Macquaker, J. H. S., and K. G. Taylor, 1996, A sequence-stratigraphic interpretation of a mudstone-dominated succession: The Lower Jurassic Cleveland Ironstone Formation, UK: Journal of the Geological Society (London), v. 153, p. 759–770.
- ↑ 56.0 56.1 56.2 Milliken, K., S.-J. Choh, P. Papazis, and J. Schieber, 2007, “Cherty” stringers in the Barnett Shale are agglutinated foraminifera: Sedimentary Geology, v. 198, p. 221–232.
- ↑ Virtasalo, J. J., L. Lowemark, H. Papunen, A. T. Kotilainen, and M. J. Whitehouse, 2010, Pyritic and baritic burrows and microbial filaments in postglacial lacustrine clays in the northern Baltic Sea: Journal of the Geological Society (London), v. 167, p. 1185–1198.
- ↑ Leonowicz, P., 2011, Sedimentation of Lower Toarcian (Lower Jurassic) brackish deposits from the Częstochowa-Wieluń region (SW Poland): Acta Geologica Polonica, v. 61, p. 215–241.
- ↑ Trabucho-Alexandre, J., R. Dirkx, H. Veld, G. Klaver, and P. L. de Boer, 2012, Toarcian black shales in the Dutch Central Graben: Record of energetic, variable depositional conditions during an oceanic anoxic event: Journal of Sedimentary Research, v. 82, no. 2, p. 104–120.
- ↑ 60.0 60.1 60.2 Milliken, K. L., and R. J. Day-Stirrat, 2013, Cementation in mudrocks: Brief review with examples from cratonic basin mudrocks, ‘’in’’ J. Chatellier, and D. Jarvie, eds., Critical assessment of shale resource plays: AAPG Memoir 103, p. 133–150.
- ↑ Könitzer, S. F., S. J. Davies, M. H. Stephenson, and M. J. Leng, 2014, Depositional controls on mudstone lithofacies in a basinal setting: Implications for the delivery of sedimentary organic matter: Journal of Sedimentary Research, v. 84, p. 198–214.
- ↑ Plint, G. A., 2014, Mud dispersal across a Cretaceous prodelta: Storm-generated, wave-enhanced sediment gravity flows inferred from mudstone microtexture and microfacies: Sedimentology, v. 61, no. 3, p. 609–647.
- ↑ 63.0 63.1 Milliken, K. L., 1994, Cathodoluminescent textures and the origin of quartz silt in Oligocene mudrocks, South Texas: Journal of Sedimentary Research, v. A64, p. 567–571.
- ↑ 64.0 64.1 Milliken, K. L., 2013, SEM-based cathodoluminescence imaging for discriminating quartz types in mudrocks: Unconventional Resources Technology Conference 1582467, 10 p.
- ↑ 65.0 65.1 65.2 Schieber, J., D. Krinsley, and L. Riciputi, 2000, Diagenetic origin of quartz silt in mudstones and implications for silica cycling: Nature, v. 406, p. 981–985.
- ↑ Schieber, J., and G. Baird, 2001, On the origin and significance of pyrite spheres in Devonian black shales of North America: Journal of Sedimentary Research, v. 71, p. 155–166.
- ↑ Schieber, J., and L. Riciputi, 2004, Pyrite ooids in Devonian black shales record intermittent sea-level drop and shallow-water conditions: Geology, v. 32, p. 305–308.
- ↑ Milliken, K. L., R. J. Day-Stirrat, P. K. Papazis, and C. Dohse, 2012b, Carbonate lithologies of the Mississippian Barnett Shale, Fort Worth Basin, Texas, ‘’in’’ J. A. Breyer, ed., Shale reservoirs—Giant resources for the 21st century: AAPG Memoir 97, p. 290–321.
- ↑ Bowles, F. A., 1968, Microstructure of sediments: Investigation with ultrathin sections: Science, v. 159, p. 1236–1237.
- ↑ 70.0 70.1 Schieber, J., and W. Zimmerle, 1998, Petrography of shales: A survey of techniques, ‘’in’’ J. Schieber, W. Zimmerle, and P. Sethi, eds., Shales and mudstones, Volume II, Stuttgart, E. Schweizerbart’sche Verlagsbuchhandlung (Nagele u. Obermiller), p. 3–12.
- ↑ McCave, I. N., and J. P. M. Syvitski, 1991, Principles and methods of geological particle size analysis, ‘’in’’ J. P. M. Syvitski, ed., Principles, methods, and application of particle size analysis: Cambridge, U.K., Cambridge University Press, 388 p.
- ↑ Bullock, P., N. Fedoroff, A. Jongerius, G. Stoops, and T. Tursina, 1985, Handbook for soil thin section description: Wolverhampton, U. K., Waine Research, 152 p.
- ↑ 73.0 73.1 73.2 Schieber, J., 1996, Early diagenetic silica deposition in algal cysts and spores: A source of sand in black shales?: Journal of Sedimentary Research, v. 66, p. 175–183.
- ↑ Milliken, K. L., W. L. Esch, R. M. Reed, and T. Zhang, 2012a, Grain assemblages and strong diagenetic overprinting in siliceous mudrocks, Barnett Shale (Mississippian), Fort Worth Basin, Texas: AAPG Bulletin, v. 96, p. 1553–1578.
- ↑ Wilson, R., and J. Schieber, 2014, Muddy prodeltaic hyperpycnites in the Lower Genesee Group of Central New York, USA: Implications for mud transport in epicontinental seas: Journal of Sedimentary Research, v. 84, p. 866–874.
- ↑ Schieber, J., and O. R. Lazar, 2004, Devonian black shales of the eastern U.S.: New insights into sedimentology and stratigraphy from the subsurface and outcrops in the Illinois and Appalachian basins: Indiana Geological Survey Open File Study 04-05, 90 p.
- ↑ Schieber, J., J. B. Southard, and K. G. Thaisen, 2007, Accretion of mudstone beds from migrating floccule ripples: Science, v. 318, p. 1760–1763.
- ↑ Loucks, R. G., and S. C. Ruppel, 2007, Mississippian Barnett Shale: Lithofacies and depositional setting of a deep-water shale-gas succession in the Fort Worth Basin, Texas: AAPG Bulletin, v. 91, no. 4, p. 579–601.
- ↑ Macquaker, J. H. S., S. J. Bentley, and K. M. Bohacs, 2010, Wave-enhanced sediment-gravity flows and mud dispersal across continental shelves: Reappraising sediment transport processes operating in ancient mudstone successions: Geology, v. 38, p. 947–950.
- ↑ Wetzel, A., and Uchman, A., 1998, Biogenic sedimentary structures in mudstones—an overview, ‘’in’’ J. Schieber, W. Zimmerle, and P. Sethi, eds., Shales and mudstones, Volume I: Stuttgart, Germany, E. Schweizerbart’sche Verlagsbuchhandlung (Nagele u. Obermiller), p. 351–369.
- ↑ 81.0 81.1 Pemberton, S. G., J. A. MacEachern, M. K. Gingras, and T. D. Saunders, 2008, Biogenic chaos: Cryptobioturbation and the work of sedimentologically friendly organisms: Palaeogeography, Palaeoclimatology, Palaeoecology, v. 270, no. 3, p. 273–279.
- ↑ Bohacs, K. M., and A. Ferrin, 2022, Monterey Formation, Miocene, California, USA—A Cenozoic biosiliceous-dominated continental slope to basin setting: A billion-barrel deep-water mudstone reservoir and source rock, in K. M. Bohacs and O. R. Lazar, eds., Sequence stratigraphy: Applications to fine-grained rocks: AAPG Memoir 126, p. 475–504.
- ↑ Bohacs, K. M., and G. J. Grabowski, 2022, Green River formation, Laney Member, Eocene, Wyoming, USA—A balanced-fill lake system with microbial carbonate and oil shale, an analog for part of the South Atlantic pre-salt, in K. M. Bohacs and O. R. Lazar, eds., Sequence stratigraphy: Applications to fine-grained rocks: AAPG Memoir 126, p. 505–536.
- ↑ Bohacs, K. M., and J. M. Guthrie, 2022, Chimney Rock Shale Member, Paradox Formation, Utah: Paleozoic, shallow carbonate-dominated shelf-to-basin billion-barrel source rocks, in K. M. Bohacs and O. R. Lazar, eds., Sequence stratigraphy: Applications to fine-grained rocks: AAPG Memoir 126, p. 223–248.
- ↑ Bohacs, K. M., O. R. Lazar, R. D. Wilson, and J. H. S. Macquaker, 2022d, Mowry Shale–Belle Fourche Shale, Bighorn Basin, Wyoming, USA—A Mesozoic clastic-biosiliceous shelf system: A prolific source rock with associated mudstone reservoir potential, in K. M. Bohacs and O. R. Lazar, eds., Sequence stratigraphy: Applications to fine-grained rocks: AAPG Memoir 126, p. 395–474.
- ↑ Bohacs, K. M., J. H. S. Macquaker, and O. R. Lazar, 2022e, Kimmeridge Clay Formation, United Kingdom—A Mesozoic clastic-carbonate shelf-to-intrashelf basin system: An outcrop-to-subsurface analog for the Haynesville, Vaca Muerta, and Bazhenov formations, in K. M. Bohacs and O. R. Lazar, eds., Sequence stratigraphy: Applications to fine-grained rocks: AAPG Memoir 126, p. 345–394.
- ↑ Campo, C., A. Morelli, A. Amorosi, L. Bruno, D. Scarponi, V. Rossi, K. M. Bohacs, and T. Drexler, 2022, Last glacial maximum depositional sequence, Po River Plain, Italy—Ultra-high resolution sequence stratigraphy of a Cenozoic coastal-plain-to-shallow-marine Foreland Basin, in K. M. Bohacs and O. R. Lazar, eds., Sequence stratigraphy: Applications to fine-grained rocks: AAPG Memoir 126, p. 537–598.
- ↑ Lazar, O. R. and J. Schieber, 2022, New Albany Shale, Illinois Basin, USA—Devonian carbonaceous mudstone accumulation in an epicratonic sea: Stratigraphic insights from outcrop and subsurface data, in K. M. Bohacs and O. R. Lazar, eds., Sequence stratigraphy: Applications to fine-grained rocks: AAPG Memoir 126, p. 249–294.
- ↑ Potma, K., R. Jonk, and K. M. Bohacs, 2022, Canol Formation, Northwest Territories, Canada—An outcrop-to-subsurface analog for the Paleozoic Horn River Shale-gas play, in K. M. Bohacs and O. R. Lazar, eds., Sequence stratigraphy: Applications to fine-grained rocks: AAPG Memoir 126, p. 295–344.
- ↑ Pye, K., and D. H. Krinsley, 1984, Petrographic examination of sedimentary rocks in the SEM using backscattered electron detectors: Journal of Sedimentary Petrology v. 54, p. 877–888.
- ↑ Trewin, N., 1988, Use of the scanning electron microscope in sedimentology, in M. Tucker, ed., Techniques in sedimentology: Oxford, U.K., Blackwell, p. 229–273.
- ↑ Camp, W. K., E. Diaz, and B. Wawak, 2013, Electron microscopy of shale hydrocarbon reservoirs: AAPG Memoir 102, 260 p.
- ↑ 93.0 93.1 Schieber, J., 2013, SEM observations on ion-milled samples of Devonian black shales from Indiana and New York: The petrographic context of multiple pore types, ‘’in’’ W. Camp, E. Diaz, and B. Wawak, eds., Electron microscopy of shale hydrocarbon reservoirs: AAPG Memoir 102, p. 153–172.
- ↑ Williams, C. J., S. P. Hesselbo, H. C. Jenkyns, and H. S. Morgans-Bell, 2001, Quartz silt in mudrocks as a key to sequence stratigraphy (Kimmeridge Clay Formation, Late Jurassic, Wessex Basin, UK): Terra Nova, v. 13, no. 6, p. 449–455.
- ↑ Erdman, N., and N. Drenzek, 2013, Integrated preparation and imaging techniques for the microstructural and geochemical characterization of shale by scanning electron microscopy, ‘’in’’ W. Camp, E. Diaz, and B. Wawak, eds., Electron microscopy of shale hydrocarbon reservoirs: AAPG Memoir 102, p. 7–14.
- ↑ Huang, J., T. Cavanaugh, and B. Nur, 2013, An introduction to SEM operational principles and geologic applications for shale hydrocarbon reservoirs, ‘’in’’ W. K. Camp, E. Diaz, and B. Wawak, eds., Electron microscopy of shale hydrocarbon reservoirs: AAPG Memoir 102, p. 1–6.
- ↑ Bollinger, D., and R. Fink, 1980, A new production technique: Ion milling: Solid-State Technology, v. 23, no. 11, p. 79–84.
- ↑ Jiang, W. T., D. R. Peacor, and E. J. Essene, 1990, Transmission electron microscopic study of coexisting pyrophyllite and muscovite—direct evidence for the metastability of illite: Clays and Clay Minerals, v. 38, p. 225–240.
- ↑ 99.0 99.1 Hover, V. C., D. R. Peacor, and L. M. Walter, 1996, STEM/AEM evidence for preservation of burial diagenetic fabrics in Devonian shales: Journal of Sedimentary Research, v. 66, p. 519–530.
- ↑ Rask, J. H., L. T. Bryndzia, N. R. Braunsdorf, and T. E. Murray, 1997, Smectite illitization in Pliocene-age Gulf of Mexico mudrocks: Clays and Clay Minerals, v. 45, p. 99–109.
- ↑ 101.0 101.1 Schieber, J., 1998c, Deposition of mudstones and shales: Overview, problems, and challenges, in J. Schieber, W. Zimmerle, and P. Sethi, eds., Shales and mudstones, Volume I: Stuttgart, E. Schweizerbart’sche Verlagsbuchhandlung (Nagele u. Obermiller), p. 131–146.
- ↑ Schieber, J., 2004, SEM and TEM study of silica diagenesis in shales from the Cretaceous Blackhawk Formation, Book Cliffs, Utah: Implications for sealing capacity: AAPG Annual Meeting, Dallas, Texas, April 18–21, 2004, Abstract Volume, p. A124.
- ↑ Tomutsa, L., D. Silin, and V. Radmilovic, 2007, Analysis of chalk petrophysical properties by means of submicron-scale pore imaging and modeling: SPE Reservoir Evaluation and Engineering, v. 10, p. 285–293.
- ↑ Loucks, R. G., R. M. Reed, S. C. Ruppel, and D. M. Jarvie, 2009, Morphology, genesis, and distribution of nanometer scale pores in siliceous mudstones of the Mississippian Barnett Shale: Journal of Sedimentary Research, v. 79, p. 848–861.
- ↑ Schieber, J., 2010, Common themes in the formation and preservation of intrinsic porosity in shales and mudstones—Illustrated with examples across the Phanerozoic: Society of Petroleum Engineers Unconventional Gas Conference, Pittsburgh, Pennsylvania, February 23–25, SPE Paper 132370, 10 p.
- ↑ Driskill, B., J. Walls, J. DeVito, and S. W. Sinclair, 2013, Applications of SEM imaging to reservoir characterization in the Eagle Ford Shale, south Texas, USA, ‘’in’’ W. Camp, E. Diaz, and B. Wawak, eds., Electron microscopy of shale hydrocarbon reservoirs: AAPG Memoir 102, p. 115–136.
- ↑ Jennings, D. S., and J. Antia, 2013, Petrographic characterization of the Eagle Ford Shale, South Texas: Mineralogy, common constituents, and distribution of nanometer-scale pore types, ‘’in’’ W. Camp, E. Diaz, and B. Wawak, eds., Electron microscopy of shale hydrocarbon reservoirs: AAPG Memoir 102, p. 101–113.
- ↑ 108.0 108.1 Schieber, J., O. R. Lazar, K. M. Bohacs, B. Klimentidis, J. Ottmann, and M. Dumitrescu, 2013, An SEM study of porosity in the Eagle Ford Shale of Texas, ‘’in’’ W. Camp, E. Diaz, and B. Wawak, eds., Electron microscopy of shale hydrocarbon reservoirs: AAPG Memoir 102, p. 42–66.
- ↑ Goldstein, J., 2003, Scanning electron microscopy and x-ray microanalysis, 3rd ed.: New York, Springer, 689 p.
- ↑ 110.0 110.1 Long, J. V. P., and S. O. Agrell, 1965, The cathodoluminescence of minerals in thin section: Mineralogical Magazine, v. 34, p. 318–326.
- ↑ Boggs, S., and D. Krinsley, 2006, Application of cathodoluminescence imaging to the study of sedimentary rocks: Cambridge, U.K., Cambridge University Press, 176 p.
- ↑ Smith, J. V., and R. C. Stenstrom, 1965, Electron-excited luminescence as a petrologic tool: Journal of Geology, v. 13, p. 627–635.
- ↑ 113.0 113.1 Zinkernagel, U., 1978, Cathodoluminescence of quartz and its application to sandstone petrology: Contributions to Sedimentology, v. 8, p. 1–69.
- ↑ Seyedolali, A., D. H. Krinsley, S. Boggs Jr., P. F. O’Hara, H. Dypvik, G. Goles, and G. Gordon, 1997, Provenance interpretation of quartz by scanning electron microscope cathodoluminescence fabric analysis: Geology, v. 25, p. 787–790.
- ↑ Schieber, J., and R. P. Wintsch, 2005, Scanned color cathodo-luminescence establishes a slate belt provenance for detrital quartz in Devonian black shales of the Appalachian basin: 15th Annual Goldschmidt Conference, Geochimica et Cosmochimica Acta, v. 10, p. A592.
- ↑ Krinsley, D., M. R. Stokes, and J. Schieber, 2009, Application of scanned color cathodoluminescence of quartz to provenance investigations of shales and mudstones: Geological Society of America Abstracts with Program, v. 41, no. 7, p. 392.
- ↑ Thyberg, B., J. Jahren, T. Winje, K. Bjørlykke, J. L. Faleide, and Ø. Marcussen, 2010, Quartz cementation in Late Cretaceous mudstones, northern North Sea: Changes in rock properties due to dissolution of smectite and precipitation of micro-quartz crystals: Marine and Petroleum Geology, v. 27, p. 1752–1764.
- ↑ Ketchum, R. A., and W. D. Carlson, 2001, Acquisition, optimization, and interpretation of x-ray computed tomographic imagery: Applications to the geosciences: Computers and Geoscience, v. 27, p. 381–400.
- ↑ Spaw, J., V. Shchelokov, and J. Milovac, 2011, Recognition of mudrock types from integration and upscaling of geologic, petrophysical and geochemical data examples from Haynesville, Woodford, and Marcellus Shales: AAPG Search and Discover article #90122, 1 p.
- ↑ Ketchum, R. A., and J. Hildebrandt, 2014, Characterizing, measuring, and utilizing the resolution of CT imagery for improved quantification of fine-scale features: Nuclear Instruments and Methods in Physics Research B, v. 324, p. 80–87.
- ↑ Spaw, J., 2015, Computed tomography (CT) scans: Frameworks for mudstone reservoir characterization: Houston Geological Society Applied Geoscience Conference Abstracts, p. 42.
- ↑ Bohacs, K. M., O. R. Lazar, and T. M. Demko, 2022a, Parasequences, in K. M. Bohacs and O. R. Lazar, eds., Sequence stratigraphy: Applications to fine-grained rocks: AAPG Memoir 126, p. 107–148.
- ↑ Udden, J. A., 1914, Mechanical composition of clastic sediments: Geological Society of America Bulletin, v. 25, p. 655–744.
- ↑ Wentworth, C. K., 1922, A scale of grade and class terms for clastic sediments: Journal of Geology, v. 30, p. 377–392.
- ↑ Krumbein, W. C., and L. L. Sloss, 1963, Stratigraphy and sedimentation, 2nd ed.: San Francisco, Freeman Press, 660 p.
- ↑ Baccelle, L., and A. Bosellini, 1965, Diagrammi per la stima visiva della composizione percentuale nelle rocce sedimentary: Annali dell’ Universita di Ferrara, Nuove Serie, Sezione IX, Scienze Geologiche e Paleontologiche, v. 1, p. 59–62.
- ↑ 127.0 127.1 Schafer, K. A. 1969, Vergleichs-Schaubilder zur Bestimmung des Allochemgehalts bioklastischer Karbonatgesteine: Neues Jahrbuch fur Geologie und Palaontologie Monatsheft, v. 1969, p. 173–184.
- ↑ Flügel, E., 1982, Microfacies analysis of limestones: Berlin, Springer, 633 p.
- ↑ Pemberton, S. G., M. Spila, A. J. Pulham, T. D. Saunders, and J. A. MacEachern, D. Robbins, I. K. Sinclair, 2001, Ichnology and sedimentology of shallow to marginal marine systems: Ben Nevis and Avalon reservoirs, Jeanne d’Arc Basin: Geological Association of Canada Short Course Notes 15, 343 p.
- ↑ Droser, M. L., and D. J. Bottjer, 1986, A semiquantitative field classification of ichnofabric: Journal of Sedimentary Research, v. 56, p. 558–559
- ↑ Droser, M. L., and D. J. Bottjer, 1988, Trends in depth and extent of bioturbation in Cambrian carbonate marine environments, western United States: Geology, v. 16, p. 233–236.
- ↑ Droser, M. L., and D. J. Bottjer, 1989, Ordovician increase in extent and depth of bioturbation: Implications for understanding early Paleozoic ecospace utilization: Geology, v. 17, p. 850–852.
- ↑ Droser, M. L., and D. J. Bottjer, 1991, Ichnofabric and basin analysis: Palaios, v. 6, p. 199–205.
- ↑ Bottjer, D. J., M. L. Droser, and D. Jablonski, 1988, Paleoenvironmental trends in the history of trace fossils: Nature, v. 333, p. 252–255.
- ↑ Bottjer, D. J., and M. L. Droser, 1992, Paleoenvironmental patterns of biogenic sedimentary structures, in C. G. Maples, and R. R. West, eds., Trace fossils: Short Courses in Paleontology v. 5, p. 130–144.
- ↑ Hasiotis, S. T., 2004, Reconnaissance of Upper Jurassic Morrison Formation ichnofossils, Rocky Mountain region, USA: Environmental, stratigraphic, and climatic significance of terrestrial and freshwater ichnocoenoses: Sedimentary Geology, v. 167, p. 177–268.
- ↑ Gerard, J., and R. Bromley, 2008, Ichnofabrics in clastic sediments: Applications to sedimentological core studies: A practical guide: Madrid, Spain, Ibergraphi 2002 SLL, 100 p.
- ↑ Bromley, R. G., 1996, Trace fossils biology, taphonomy and applications, 2nd ed.: London, Chapman and Hall, 361 p.
- ↑ Brett, C. E., and P. A. Allison, 1998, Paleontological approaches to the environmental interpretation of marine mudrocks, in J. Schieber, W. Zimmerle, and P. Sethi, eds., Shales and mudstones I.: Stuttgart, Germany, E. Schweizerbart’sche Verlagsbuchhandlung (Nagele u. Obermiller), p. 301–349.
- ↑ 140.0 140.1 140.2 140.3 Potter, P. E., J. B. Maynard, and P. J. Depetris, 2005, Mud and mudstones: Introduction and overview: Berlin, Springer-Verlag, 297 p.
- ↑ Hinshelwood, C. N., (quoted in M. J. Nye), 1993, From chemical philosophy to theoretical chemistry: Oakland, California, University of California Press, 356 p.
- ↑ 142.0 142.1 142.2 Soil Survey Division Staff, 2014, Assessing carbonates in the field with a Dilute Hydrochloric Acid (HCl) Solution: USDA Soil Survey Technical Note 5.
- ↑ 143.0 143.1 Bohacs, K. M., O. R. Lazar, and J. D. Ottman, 2022c, Parasequence sets and depositional sequences, in K. M. Bohacs and O. R. Lazar, eds., Sequence stratigraphy: Applications to fine-grained rocks: AAPG Memoir 126, p. 149–194.
- ↑ Reid, W. P., 1969, Mineral staining tests: Colorado School of Mines Mineral Industries Bulletin, v. 12, no. 3, p. 1–20.
- ↑ 145.0 145.1 Friedman, G. M., C. A. Sternback, and A. Walton, 2007, Identification of minerals by staining, ‘’in’’ J. D. Walker, and A. A. Cohen, ed., The geoscience handbook, 4th ed.: Washington, DC, American Geological Institute, p. 125–132.
- ↑ Evamy, B. D., 1969, The precipitational environment and correlation of some calcite cements deduced from artificial staining: Journal of Sedimentary Petrology, v. 39, p. 787–792.
- ↑ Dickson, J. A. D., 1965, A modified staining technique for carbonates in thin section: Nature, v. 205, p. 587.
- ↑ Dickson, J. A. D., 1966, Carbonate identification and genesis as revealed by staining: Journal of Sedimentary Petrography v. 36, p. 491–505.
- ↑ Schneidermann, N., and P. A. Sandberg, 1971, Calcite-aragonite differentiation by selective staining and scanning electron microscopy: Transactions of the Gulf Coast Association of Geological Societies, v. 21, p. 349–351.
- ↑ 150.0 150.1 Pratt, L. M., 1984, Influence of paleonvironmental factors on preservation of organic matter in Middle Cretaceous Greenhorn Formation, Pueblo, Colorado: AAPG Bulletin, v. 68, p. 1146–1159.
- ↑ 151.0 151.1 Jones, B., and D. A. C. Manning, 1994, Comparison of geochemical indices used for the interpretation of palaeoredox conditions in ancient mudstones: Chemical Geology, v. 111, p. 111–129.
- ↑ 152.0 152.1 Bertrand, P., G. Shimmield, P. Martinez, F. Grousset, F. Jorissen, M. Paterne, C. Pujol, I. Bouloubassi, P. B. Menard, and J. P. Peypouquet, 1996, The glacial ocean productivity hypothesis: The importance of regional temporal and spatial studies: Marine Geology, v. 130, p. 1–9.
- ↑ 153.0 153.1 Dean, W. E., and M. A. Arthur, 1998, Geochemical expressions of cyclicity in Cretaceous pelagic limestone sequences: Niobrara Formation, Western Interior Seaway, ‘’in’’ W. E. Dean and M. A. Arthur, eds., Stratigraphy and paleoenvironments of the Cretaceous Western Interior Seaway, USA: SEPM Concepts in Sedimentology and Paleontology 6, p. 227–255.
- ↑ 154.0 154.1 154.2 Murphy, A. E., B. B. Sageman, and D. J. Hollander, 2000a, Eutrophication by decoupling of the marine biogeochemical cycles of C, N, and P: A mechanism for the Late Devonian mass extinction: Geology, v. 28, p. 427–430.
- ↑ 155.0 155.1 155.2 Murphy, A. E., B. B. Sageman, D. J. Hollander, T. W. Lyons, and C. E. Brett, 2000b, Black shale deposition and faunal overturn in the Devonian Appalachian basin: Clastic starvation, seasonal water-column mixing, and efficient biolimiting nutrient recycling: Paleoceanography, v. 15, p. 280–291.
- ↑ 156.0 156.1 156.2 156.3 Werne, J. P., B. B. Sageman, W. W. Lyons, and D. J. Hollander, 2002, An integrated assessment of a “type euxinic” deposit: Evidence for multiple controls on black shale deposition in the Middle Devonian Oatka Creek Formation: Journal of Science, v. 302, p. 110–143.
- ↑ Lazar, O., and J. Schieber, 2003, The influence of sedimentary processes on element distribution in the Devonian New Albany Shale of the Illinois Basin: Geological Society of America Abstract with Program, v. 35, no. 6, p. 83.
- ↑ 158.0 158.1 Lyons, T. W., J. P. Werne, D. J. Hollander, and R. W. Murray, 2003, Contrasting sulfur geochemistry and Fe/Al and Mo/Al ratios across the last oxic-to-anoxic transition in the Cariaco Basin, Venezuela: Chemical Geology, v. 195, p. 131–157.
- ↑ 159.0 159.1 159.2 159.3 Sageman, B. B., A. E. Murphy, J. P. Werne, C. A. Ver Straeten, D. J. Hollander, and T. W. Lyons, 2003, A tale of shales: The relative roles of production, decomposition, and dilution in the accumulation of organic-rich strata, Middle-Upper Devonian, Appalachian basin: Chemical Geology, v. 195, p. 229–273.
- ↑ Algeo, T. J., 2004, Can marine anoxic events draw down the trace element inventory of seawater?: Geology, v. 32, p. 1057–1060.
- ↑ Rimmer, S. M., 2004, Geochemical paleoredox indicators in Devonian-Mississippian black shales, Central Appalachian Basin (USA): Chemical Geology, v. 206, p. 373–391.
- ↑ 162.0 162.1 162.2 162.3 162.4 Rimmer, S. M., J. A. Thompson, S. A. Goodnight, and T. L. Robl, 2004, Multiple controls on the preservation of organic matter in Devonian-Mississippian marine black shales: Geochemical and petrographic evidence: Palaeogeography, Palaeoclimatology, Palaeoecology, v. 215, p. 125–154.
- ↑ Algeo, T. J., and T. W. Lyons, 2006, Mo–total organic carbon covariation in modern anoxic marine environments: Implications for analysis of paleoredox and paleohydrographic conditions: Paleogeography, v. 21, p. PA1016.
- ↑ Tribovillard, N., T. J. Algeo, T. Lyons, and A. Riboulleau, 2006, Trace metals as paleoredox and paleoproductivity proxies: An update: Chemical Geology, v. 232, p. 12–32.
- ↑ Algeo, T. J., and Maynard, J. B., 2008, Trace-metal covariation as a guide to water-mass conditions in ancient anoxic marine environments: Geosphere, v. 4, no. 5, p. 872–887.
- ↑ Rowe, H., N. Hughes, and K. Robinson, 2012, The quantification and application of handheld energy-dispersive x-ray fluorescence (ED-XRF) in mudrock chemostratigraphy and geochemistry: Chemical Geology, v. 324–325, p. 122–131.
- ↑ Norrish, K., and B. W. Chappell, 1977, X-ray fluorescence spectrometry, ‘’in’’ J. Zussman, ed., Physical methods of determinative mineralogy: London, Academic Press, p. 201–271.
- ↑ Tertian, R., and F. Claisse, 1982, Principles of quantitative x-ray fluorescence analysis: London, Heyden, p. 385.
- ↑ Fairchild, I., G. Hendry, M. Quest, and M. Tucker, 1988, Chemical analysis of sedimentary rocks, ‘’in‘’ M. E. Tucker, ed., Techniques in sedimentology: Oxford, U.K., Blackwell Scientific, p. 274–354.
- ↑ Rollinson, H., 1993, Using geochemical data: Evaluation, presentation, interpretation: New York, John Wiley, 384 p.
- ↑ 171.0 171.1 Fitton, G., 1997, X-Ray fluorescence spectrometry, ‘’in’’ R. Gill, ed., Modern analytical geochemistry: An introduction to quantitative chemical analysis techniques for earth, environmental, and material scientists: Harlow, U.K., Addison Wesley Longman, p. 87–115.
- ↑ Jansen, J. H. F., S. J. Van der Gaast, B. Koster, and A. J. Vaars, 1998, CORTEX, a shipboard XRF-scanner for element analyses in split sediment cores: Marine Geology, v. 151, p. 143–153.
- ↑ Rothwell, R. G., B. Hoogakker, J. Thomson, I. W. Croudace, and M. Frenz, 2006, Turbidite emplacement on the southern Balearic Abyssal Plain (western Mediterranean Sea) during marine isotope stages 1-3: An application of IT-RAX XRF scanning of sediment cores to lithostratigraphic analysis, ‘’in’’ R. G. Rothwell, ed., New techniques in sediment core analysis: Geological Society (London) Special Publication 267, p. 79–98.
- ↑ Banerjee, S., 2011, Biogeochemical evolution of the Western Interior Basin of North America during a Kasimovian Highstand and Regression, M.Sc. thesis, Texas A&M University, College Station, Texas, 55 p.
- ↑ Liu, X., S. M. Colman, E. T. Brown, E. C. Minor, and H. Li, 2013, Estimation of carbonate, total organic matter, and biogenic silica content by FTIR and XRF techniques in lacustrine sediments: Journal of Paleolimnology, v. 50, p. 387–398.
- ↑ Adams, J. A. S., and C. E. Weaver, 1958, Thorium-to-uranium ratios as indicators of sedimentary processes: Example of concept of geochemical facies: AAPG Bulletin, v. 42, p. 387–430.
- ↑ Berner, R. A., 1969, The synthesis of framboidal pyrite: Economic Geology, v. 64, p. 383–384.
- ↑ Berner, R. A., 1970, Sedimentary pyrite formation: American Journal of Science, v. 268, p. 1–23.
- ↑ Berner, R. A., 1980, Early diagenesis: A theoretical approach: Princeton Series in Geochemistry 2, 237 p.
- ↑ Berner, R. A., 1981, A new geochemical classification of sedimentary environments: Journal of Sedimentary Petrology, v. 51, p. 359–366.
- ↑ Berner, R. A., 1982, Burial of organic carbon and pyrite sulfur in the modern ocean: Its geochemical and environmental significance: American Journal of Science, v. 282, p. 451–473.
- ↑ Ernst, W., 1970, Geochemical facies analysis: New York, Elsevier, 152 p.
- ↑ Lewan, M. D., and J. B. Maynard, 1982, Factors controlling enrichment of vanadium and nickel in the bitumen of organic sedimentary rocks: Geochimica et Cosmochimica Acta, v. 46, p. 2547–2560.
- ↑ Dypvik, H., 1984, Geochemical compositions and depositional conditions of Upper Jurassic and Lower Cretaceous Yorkshire clays, England: Geological Magazine, v. 121, p. 489–504.
- ↑ Lewan, M. D., 1984, Factors controlling the proportionality of vanadium to nickel in crude oils: Geochimica et Cosmochimica Acta, v. 48, p. 2231–2238.
- ↑ Raiswell, R., R. A. Berner, 1985, Pyrite formation in euxinic and semi-euxinic sediments: American Journal of Science, v. 285, p. 710–724.
- ↑ Dill, H., 1986, Metallogenesis of early Paleozoic graptolite shales from the Graefenthal Horst (Northern Bavaria, Federal Republic of Germany): Economic Geology, v. 81, p. 889–903.
- ↑ Calvert, S. E., 1987, Oceanographic controls on the accumulation of organic matter in marine sediments, ‘’in’’ J. Brooks and A. J. Fleet, eds., Marine petroleum source rocks: Geological Society (London) Special Publication 26, p. 137–151.
- ↑ Raiswell, R., F. Buckley, R. A. Berner, and T. F. Anderson, 1988, Degree of pyritization of iron as a paleoenvironmental indicator of bottom-water oxygenation: Journal of Sedimentary Petrology, v. 58, p. 812–819.
- ↑ Canfield, D. E., 1989, Sulfate reduction and oxic respiration in marine sediments: Implications for organic-carbon preservation in euxinic environments: Deep-Sea Research, v. 36, p. 121–138.
- ↑ Dean, W. E., and M. A. Arthur, 1989, Iron-sulfur-carbon relationships in organic-carbon-rich sequences I: Cretaceous Western Interior Seaway: American Journal of Science, v. 289, p. 708–743.
- ↑ Calvert, S. E., and R. E. Karlin, 1991, Relationships between sulphur, organic carbon, and iron in the modern sediments of the Black Sea: Geochimica et Cosmochimica Acta, v. 55, p. 2483–2490.
- ↑ Calvert, S. E., and T. F. Pedersen, 1992, Organic carbon accumulation and preservation in marine sediments: How important is the anoxia? ‘’in’’ J. K. Whelan, and J. W. Farrington, eds., Organic matter: Productivity, accumulation and preservation in recent and ancient sediments: New York, Columbia University Press, p. 231–263.
- ↑ Calvert, S. E., and T. F. Pedersen, 1993, Geochemistry of Recent oxic and anoxic marine sediments: Implications for the geologic record: Marine Geology, v. 113, p. 67–88.
- ↑ Arthur, M. A., and B. B. Sageman, 1994, Marine black shales: Depositional mechanisms and environments of ancient deposits: Annual Review of Earth and Planetary Sciences, v. 22, p. 499–551.
- ↑ Wignall, P. B., 1994, Black shales: Oxford, Clarendon Press, p. 127.
- ↑ Isaacs, C. M., 1981, Field characterization of rocks in the in the Monterey formation examined laterally along the Santa Barbara Coast, California, in C. M. Isaacs, ed., Guide to the Monterey Formation in the California coastal area, Ventura to San Luis Obispo: AAPG Guidebook 52, p. 39–52.
- ↑ 198.0 198.1 198.2 198.3 Schwalbach, J. R., and K. M. Bohacs, 1992, Sequence stratigraphy in fine-grained rocks: Examples from the Monterey Formation: Los Angeles, SEPM—the Pacific Section, 80 p.
- ↑ Hay, W. W., 1995, Paleoceanography of marine organic-carbon-rich sediments, ‘’in’’ A.-Y. Huc, ed., Paleogeography, paleoclimate, and source rocks: AAPG Studies in Geology 40, p. 21–59.
- ↑ Rühlemann, C., P. J. Mueller, and R. R. Schneider, 1999, Organic carbon and carbonate as paleoproductivity proxies: Examples from high and low productivity areas of the tropical Atlantic, Uses of proxies in paleoceanography: Examples from the south Atlantic: Berlin, Springer-Verlag, p. 315–344.
- ↑ Wortmann, U. G., R. Hesse, and W. Zacher, 1999, Major-element analysis of cyclic black shales: Paleoceanographic implications for the Early Cretaceous deep western Tethys: Paleoceanography, v. 14, p. 525–541.
- ↑ Tribovillard, N., A. Ramdani, and A. Trentesaux, 2005, Controls on organic accumulation in Upper Jurassic shales of northwestern Europe as inferred from trace-metal geochemistry, in N. B. Harris, ed., The deposition of organic-carbon-rich sediments: Models, mechanisms, and consequences: SEPM Special Publication 82, p. 145–164.
- ↑ Hardy, R., and M. Tucker, 1988, X-ray powder diffraction of sediments, ‘’in’’ M. Tucker,ed., Techniques in sedimentology: Oxford, U.K., Blackwell, p. 191–228.
- ↑ Bish, D. L., and J. E. Post, 1989, Modern powder diffraction: Mineralogical Society of America, Reviews in Mineralogy, v. 20, p. 369.
- ↑ Mumme, W. G., G. Tsambourakis, I. C. Madsen, and R. J. Hill, 1996, Improved petrological modal analyses from x-ray powder diffraction data by use of the Rietveld method. Part II. Selected sedimentary rocks: Journal of Sedimentary Research, v. 66, p. 132–138.
- ↑ Moore, D. M., and R. C. Reynolds Jr., 1997, X-ray diffraction and the identification and analysis of clay minerals, 2nd ed.: New York, Oxford University Press, 400 p.
- ↑ 207.0 207.1 207.2 Tyson, R. V., 1995, Sedimentary organic matter: Organic facies and palynofacies: Amsterdam, the Netherlands, Springer, 615 p.
- ↑ Tyson, R. V., 2001, Sedimentation rate, dilution, preservation and total organic carbon: Some results of a modelling study: Organic Geochemistry, v. 32, p. 333–339.
- ↑ Tyson, R. V., 2005, The “productivity versus preservation” controversy: Cause, flaws, and resolution, ‘’in’’ N. B. Harris, ed., The deposition of organic-carbon-rich sediments: Models, mechanisms, and consequences: SEPM Special Publication 82, p. 17–33.
- ↑ 210.0 210.1 210.2 210.3 Bohacs, K. M., A. R. Carroll, J. E. Neal, and P. J. Mankiewicz, 2000, Lake-basin type, source potential, and hydrocarbon character: An integrated sequence-stratigraphic-geochemical framework, in E. Gierlowski-Kordesch, and K. Kelts, eds., Lake basins through space and time: AAPG Studies in Geology 46, p. 3–37.
- ↑ Harris, N. B., 2005, The deposition of organic-carbon-rich sediments: Models, mechanisms, and consequences-introduction, in N. B. Harris, ed., The deposition of organic-carbon-rich sediments: Models, mechanisms, and consequences: SEPM Special Publication 82, p. 1–5.
- ↑ Katz, B. J., 2005, Controlling factors on source rock development-a review of productivity, preservation, and sedimentation rate, in N. B. Harris, ed., The deposition of organic-carbon-rich sediments: Models, mechanisms, and consequences: SEPM Special Publication 82, p. 7–16.
- ↑ 213.0 213.1 Bohacs, K. M., O. R. Lazar, T. M. Demko, J. Ottmann, and K. Potma, 2022b, Sequence sets and composite sequences, in K. M. Bohacs and O. R. Lazar, eds., Sequence stratigraphy: Applications to fine-grained rocks: AAPG Memoir 126, p. 195–222.
- ↑ Creaney, S., and Q. R. Passey, 1993, Recurring patterns of total organic carbon and source rock quality within a sequence stratigraphic framework: AAPG Bulletin, v. 77, p. 386–401.
- ↑ 215.0 215.1 Peters, K. E., and M. R. Cassa, 1994, Applied source rock geochemistry, in L. B. Magoon, and W. G. Dow, eds., The Petroleum system—From source to trap: AAPG Memoir 60, p. 93–120.
- ↑ 216.0 216.1 216.2 216.3 216.4 Peters, K. E., C. C. Walters, and J. M. Moldowan, 2005, The biomarker guide: Biomarkers and isotopes in the environment and human history, 2nd ed.: Cambridge, U.K., Cambridge University Press, 1155 p.
- ↑ 217.0 217.1 Cite error: Invalid
<ref>
tag; no text was provided for refs namedCrnyPssy1993
- ↑ Bohacs, K. M., O. R. Lazar, J. Ottmann, K. Potma, and T. M. Demko, 2013, Shale-gas-reservoir families—translating sequence stratigraphy into robust predictions of reservoir distribution and potential: AAPG Search and Discovery article #90163.
- ↑ Espitalié, J., G. Deroo, and F. Marquis, 1985, La pyrolyse Rock-Eval et ses applications: Review Institut Français du Pétrole, Partie 1 and 2, v. 40, p. 563–784, Partie 3, v. 41, p. 73–89.
- ↑ Espitalié, J., J. L. Laporte, M. Madec, F. Marquis, P. Lepat, J. Paulet, and A. Boutefeu, 1977, Méthode rapide de caractérisation des roches mères, de leur potentiel petrolier et leur degré d’évolution: Review Institut Français du Pétrole, v. 32, p. 23–42.
- ↑ Lafargue, E., F. Marquis, and D. Pillot, 1998, Rock-Eval 6 applications in hydrocarbon exploration, production, and soil contamination studies: Institut Français du Pétrole, v. 53, p. 421–437.
- ↑ Peters, K. E., 1986, Guidelines for evaluating petroleum source rock using programmed pyrolysis: AAPG Bulletin, v. 70, p. 329.
- ↑ 223.0 223.1 Tissot, B. P., and D. H. Welte, 1984, Petroleum formation and occurrence: Berlin, Springer-Verlag, 699 p.
- ↑ Hunt, J. M., 1979, Petroleum geochemistry and geology: San Francisco, W. H. Freeman, 617 p.
- ↑ 225.0 225.1 Durand, B., 1980, Sedimentary organic matter and kerogen: Definition and quantitative importance of kerogen, in B. Durand, ed., Kerogen, insoluble organic matter from sedimentary rocks: Paris, Editions Technip, p. 13–34.
- ↑ 226.0 226.1 Vandenbroucke, M., and C. Largeau, 2007, Kerogen origin, evolution and structure: Organic Geochemistry, v. 38, p. 719–833.
- ↑ 227.0 227.1 Carroll, A. R., and K. M. Bohacs, 1999, Stratigraphic classification of ancient lakes: Balancing tectonic and climatic controls: Geology, v. 27, no. 2, p. 99–102.
- ↑ 228.0 228.1 Carroll, A. R., and K. M. Bohacs, 2001, Lake type controls on hydrocarbon source rock potential in nonmarine basins: AAPG Bulletin, v. 85, p. 1033–1053.
- ↑ Mello, M. R., E. A. M. Koutsoukos, E. V. Santos Neto, and A. C. Silva Telles Jr., 1993, Geochemical and micropaleontological characterization of lacustrine and marine hypersaline environments from Brazilian sedimentary basins, in B. J. Katz, and L. M. Pratt, ed., Source rocks in a sequence stratigraphic framework: AAPG Studies in Geology 37, p. 17–34.
- ↑ Isaksen, G. H., and K. M. Bohacs, 1995, Geological controls of source rock geochemistry through relative sea level; Triassic, Barents Sea, in B. J. Katz, ed., Petroleum source rocks: Berlin, Springer Verlag, p. 25–50.
- ↑ Philp, R. P., and T. D. Gilbert, 1986, The detection and identification of biological markers by computerized-gas chromatography-mass spectrometry, in R. B. Johns, ed., Biological markers in the sedimentary record: Amsterdam, the Netherlands, Elsevier, p. 227–248.
- ↑ Philp, R. P., 1993, Oil-oil and oil-source rock correlations: Techniques, in M. H. Engel, and S. A. Macko, eds., Organic geochemistry: Principles and applications: New York, Plenum Press, p. 445–460.
- ↑ Schouten, S., M. R. B. de Loureiro, J. S. Sinninghe Damsté, J. W. de Leeuw, and J. W., 2001, Molecular biogeochemistry of Monterey sediments, Naples Beach, California. I: Distribution of hydrocarbons and organic sulfur compounds, in C. M. Isaacs, and J. Rullkötter, eds., The Monterey Formation: From rocks to molecules: New York, Columbia University Press, p. 150–174.
- ↑ Rowland, S. J., and J. R. Maxwell, 1984, Reworked triterpenoid and steroid hydrocarbons in a recent sediment: Geochimica Cosmochimca Acta, v. 48, p. 617–624.
- ↑ Farrimond, P., G. Eglinton, S. C. Brassell, and H. C. Jenkyns, 1989, Toarcian anoxic event in Europe: An organic geochemical study: Marine and Petroleum Geology, v. 6, p. 136–147.
- ↑ 236.0 236.1 Faure, G., 1986, Principles of isotope geology, 2nd ed.: Hoboken, New Jersey, John Wiley & Sons, 589 p.
- ↑ 237.0 237.1 237.2 Hoefs, J., 1997, Stable isotope geochemistry, 4th ed.: Berlin, Springer-Verlag, 201 p.
- ↑ Brownlow, A. H., 1996, Geochemistry, 2nd ed.: Englewood-Cliffs, New Jersey, Prentice-Hall, 580 p.
- ↑ Klein, J. S., P. Mozley, A. Campbell, and R. Cole, 1999, Spatial distribution of carbon and oxygen isotopes in laterally extensive carbonate-cemented layers: Implications for mode of growth and subsurface identification: Journal of Sedimentary Research, v. 69, p. 184–191.
- ↑ Veizer, J., W. T. Holser, and C. K. Wilgus, 1980, Correlation of 13C/12C and 34S/32S secular variations: Geochemica et Cosmochimica Acta, v. 44, p. 579–587.
- ↑ Joachimski, M. M., and W. Buggisch, 1993, Anoxic events in the Late Frasnian—causes of the Frasnian–Famennian Faunal crisis: Geology, v. 21, p. 675–678.
- ↑ Jenkyns, H. C., A. S. Gale, and R. M. Corfield, 1994, Carbon- and oxygen-isotope stratigraphy of the English Chalk and Italian Scaglia and its palaeoclimatic significance: Geological Magazine, v. 131, p. 1–34.
- ↑ Menegatti, A. P., H. Weissert, R. S. Brown, R. V. Tyson, P. Farrimond, A. Strasser, and M. Caron, 1998, High-resolution δ13C stratigraphy through the early Aptian “Livello Selli” of the Alpine Tethys: Paleoceanography, v. 13, p. 530–545.
- ↑ Jenkyns, H., and P. A. Wilson, 1999, Stratigraphy, paleoceanography and evolution of Cretaceous Pacific guyots: Relics from a greenhouse earth: American Journal of Science, v. 299, p. 341–392.
- ↑ Kump, L. R., and M. A. Arthur, 1999, Interpreting carbon-isotope excursions: Carbonates and organic matter: Chemical Geology, v. 161, p. 181–198.
- ↑ Danelian, T., H. Tsikos, S. Gardin, F. Baudin, J. P. Bellier, and L. Emmanuel, 2004, Global and regional palaeoceanographic changes as recorded in the mid-Cretaceous (Aptian-Albian) sequence of the Ionian zone (NW Greece): Journal of the Geological Society, v.161, p. 703–709.
- ↑ Buggisch, W., and M. M. Joachimski, 2006, Carbon isotope stratigraphy of the Devonian of central and southern Europe: Palaeogeography, Palaeoclimatology, Palaeoecology, v. 240, p. 68–88.
- ↑ Dumitrescu, M., and S. C. Brassell, 2006, Compositional and isotopic characteristics of organic matter for the early Aptian oceanic anoxic event at Shatsky Rise, ODP Leg 198: Palaeogeography, Palaeoclimatology, Palaeoecology, v. 235, p. 168–191.
- ↑ 249.0 249.1 Lonstaffe, F., 2003, Isotopic methods in sedimentology, in G. V. Middleton, ed., Encyclopedia of sediments and sedimentary rocks: Dordrecht, Germany, Kluwer Academic, p. 385–397.
- ↑ Hudson, J. D., 1977, Stable isotopes and limestone lithification: Journal of the Geological Society (London), v. 133, p. 637–660.
- ↑ Joachimski, M. M., R. van Geldern, S. Breisig, W. Buggisch, and J. Day, 2003, Oxygen isotope evolution of biogenic calcite and apatite during the Middle and Late Devonian: International Journal of Earth Sciences, v. 93, p. 542–553.
- ↑ 252.0 252.1 Machent, P. G., K. G. Taylor, J. H. S. Macquaker, and J. D. Marshall, 2007, Patterns of early post-depositional and burial cementation in distal shallow-marine sandstones: Upper Cretaceous Kenilworth Member, Book Cliffs, Utah, USA: Sedimentary Geology, v. 198, p. 125–145.
- ↑ 253.0 253.1 Machent, P. G., K. G. Taylor, J. H. S. Macquaker, and J. D. Marshall, 2012, Distribution and petrography of concretionary carbonate in falling-stage, delta-front sandstone succession: Upper Cretaceous Panther Tongue Member, Book Cliffs, Utah, in S. Morad, M. Ketzer, and L. F. De Ros, eds., Linking diagenesis to sequence stratigraphy of sandstone rocks: IAS Special Publication, v. 45, p. 99–123.
- ↑ Schidlowski, M., J. M. Hayes, and I. R. Kaplan, 1983, Isotopic inferences of ancient biochemistries, in J. W. Schopf, ed., Earth’s earliest biosphere: Princeton, New Jersey, Princeton University Press, p. 149–186.
- ↑ Irwin, H., C. Curtis, and M. Coleman, 1977, Isotopic evidence for source of diagenetic carbonates formed during burial of organic-rich sediments: Nature, v. 269, p. 209–213.
- ↑ Smith, B. N., and S. Epstein, 1971, Two categories of 13C/12C ratios for higher plants: Plant Physiology, v. 47, p. 380–384.
- ↑ Stuiver, M., 1978, Atmospheric carbon dioxide and carbon reservoir changes: Science, v. 199, p. 253–258.
- ↑ Farquhar, G. D., 1980, Carbon isotope discrimination by plants: Effects of carbon dioxide concentration and temperature via the ratio of intercellular and atmospheric CO2 concentrations, in G. L. Pearman, ed., Carbon dioxide and climate: Australian Research, Australian Academy of Science, p. 105–110.
- ↑ Farquhar, G. D., 1983, On the nature of carbon isotope discrimination in C4 species: Australian Journal of Plant Physiology, v. 10, p. 205–226.
- ↑ O’Leary, M. H., 1981, Carbon isotope fractionation in plants: Phytochemistry, v. 20, p. 553–567.
- ↑ Schidlowski, M., 1988, A 3800-million-year isotopic record of life from carbon in sedimentary rocks: Nature, v. 333, p. 313–318.
- ↑ Fogel, M. L., and L. A. Cifuentes, 1993, Isotope fractionation during primary production, in M. H. Engel and S. A. Macko, eds., Organic geochemistry: Principles and applications: New York, Plenum Press, p. 73–98.
- ↑ Killops, S., and V. Killops, 2005, Introduction to organic geochemistry: New York, Blackwell, 393 p.
- ↑ Coplen, T. B., 1996, New guidelines for reporting stable hydrogen, carbon, and oxygen isotope-ratio data: Geochimica et Cosmochimica Acta v. 60, p. 3359–3360.
- ↑ Werner, R. A., and W. A. Brand, 2001, Referencing strategies and techniques in stable isotope ratio analysis: Rapid Communications in Mass Spectrometry, v. 15, p. 501–519.
- ↑ Pike, B., and R. Duey, 2002, Logging history rich with innovation: Hart’s E&P, September 2002, p. 52–55.
- ↑ 267.0 267.1 Serra, O., 1984, Fundamentals of well-log interpretation: Developments in Petroleum Science, v. 15, Part A, p. iii–vii, 1–423.
- ↑ 268.0 268.1 Schlumberger Limited, 1991, Log interpretation principles/applications: Houston, Texas, Schlumberger Educational Services, 142 p.
- ↑ 269.0 269.1 Emery, D., and K. J. Myers, 1996, Sequence stratigraphy: Oxford, Blackwell Science, 297 p.
- ↑ 270.0 270.1 Rider, M., 2002, The geological interpretation of well logs: Marsa, Malta, Interprint, 280 p.
- ↑ 271.0 271.1 Evenick, J. C., 2008, Introduction to well logs and subsurface maps: Tulsa, Oklahoma, PennWell, 236 p.
- ↑ Passey, Q. R., S. Creaney, J. B. Kulla, F. J. Moretti, and J. D. Stroud, 1990, A practical model for organic richness from porosity and resistivity logs: AAPG Bulletin, v. 74, p. 1777–1794.
- ↑ Bohacs, K. M., and K. Miskell-Gerhardt, 1998, Well-log expression of lake strata; controls of lake-basin type and provenance, contrasts with marine strata: AAPG Search and Discovery article #90937.
- ↑ 274.0 274.1 Bohacs, K. M., G. J. Grabowski Jr., A. R. Carroll, and K. J. Miskell-Gerhardt, 2001, Non-marine sequence stratigraphy field workshop: Guidebook to Wyoming and Colorado outcrops: Denver, Colorado, SEPM Rocky Mountain Section, 102 p.
- ↑ Martel, T., 2013, Pitfalls in assessing lacustrine shale versus marine shale prospects: AAPG Search and Discovery article #41188, 29 p.
- ↑ Bessereau, G., and F. Guillocheau, 1995, Stratgraphie sequentielle et distribution de la matiere organique dans le Lias du bassin de Paris: Comptes Rendu de l’Académie des Sciences, v. 316, p. 1271–1278.
- ↑ Spears, R. W., and S. L. Jackson, 2009, Development of a predictive tool for estimating well performance in horizontal shale gas wells in the Barnett Shale, North Texas, USA: Petrophysics, v. 50, p. 19–31.
- ↑ 278.0 278.1 Wheeler, H. E., 1964, Base level, lithosphere surface, and time-stratigraphy: Geological Society of America, Bulletin, v. 75, p. 599–610.
- ↑ Payton, C. E., 1977, Seismic stratigraphy-applications to hydrocarbon exploration: AAPG Memoir 26, 516 p.
- ↑ 280.0 280.1 Sheriff, R. E., 1980, Seismic stratigraphy: Boston, IHRDC, 227 p.
- ↑ 281.0 281.1 281.2 281.3 281.4 281.5 281.6 281.7 Abreu, V., J. Neal, K. M. Bohacs, and J. L. Kalbas, 2010, Sequence stratigraphy of siliciclastic systems—the ExxonMobil methodology: Atlas of exercises: SEPM Concepts in Sedimentology and Paleontology 9, 226 p.
- ↑ 282.0 282.1 Veeken, P. C. H., and B. van Moerkerken, 2013, Seismic stratigraphy and depositional facies models: New York, Academic Press, 442 p.
- ↑ 283.0 283.1 283.2 283.3 Mitchum Jr., R. M., and P. R. Vail, 1977, Seismic stratigraphy and global changes of sea-level, Part 7: Stratigraphic interpretation of seismic reflection patterns in depositional sequences, in C. E. Payton, ed., Seismic Stratigraphy—applications to hydrocarbon exploration, AAPG Memoir 26, p. 135–144.
- ↑ 284.0 284.1 284.2 284.3 284.4 284.5 284.6 284.7 Mitchum, R. M., P. R. Vail, and J. B. Sangree, 1977, Seismic stratigraphy and global changes of sea level, Part 6: Stratigraphic interpretation of seismic reflection patterns in depositional sequences: Section 2. Application of seismic reflection configuration to stratigraphic interpretation, in C. E. Payton, ed., Seismic stratigraphy applications to hydrocarbon exploration: AAPG Memoir 26, p. 117–133.
- ↑ 285.0 285.1 285.2 285.3 285.4 Ramsayer, G. R., 1979, Seismic stratigraphy, a fundamental exploration tool: 11th Annual Offshore Technology Conference Proceedings, Houston, Texas, 1 May, 1979, p. 101–109.
- ↑ Armentrout, J. M., S. J. Malecek, L. B. Fearn, C. E. Sheppard, P. H. Naylor, A. W. Miles, R. J. Demarais, and R. J. Dunay, 1993, Log motif analysis of Paleogene depositional systems tracts, central and northern North Sea: Defined by sequence analysis, in J. R. Parker, ed., Petroleum geology of northwest Europe: Proceedings of 4th Conference, London, 29 March–1 April 1992, p. 45–57.
- ↑ 287.0 287.1 287.2 287.3 Campbell, C. V., 1967, Lamina, laminaset, bed and bedset: Sedimentology, v. 8, p. 7–26.
- ↑ 288.0 288.1 Gao, D., 2011, Latest developments in seismic texture analysis for subsurface structure, facies and reservoir characterization: A review: Geophysics v. 76, p. W1–W13.
- ↑ Garfield, T. R., 2000, New insight into the three-dimensional architecture of deep-water facies: The product of a multi-disciplinary approach, ‘’in’’ T. R. Garfield, R. T. Beaubouef, and D.K. Sickafoose, eds., High-resolution sequence stratigraphy: A tool for improved reservoir delineation in passive margin settings—Examples from the divergent margin of the South Atlantic Salt Basin: 31st International Geological Congress Abstracts, CD.
- ↑ Vinther, R., K. Mosegaard, K. Kierkegaard, I. Abatzis, C. Andersen, and F. If, 1995, Seismic texture classification: A computer-aided approach to stratigraphic analysis, in SEG 65th Annual International Meeting Technical Program Expanded Abstracts, Houston, Texas, October 8–13, 1995, p. 153–155.
- ↑ DeGroot, P., 1999, Volume transformation by way of neural network mapping: EAGE 61st Conference, Helsinki, Finland, June 7–11, paper 3-37, 5 p.
- ↑ West, B. P., and S. R. May, 2003, A method for training a probabilistic neural network to map seismic attributes or similar quantities: U.S. Patent No. 192467 filed on 2002-07-10.
- ↑ Marroquin, I. D., J.-J. Brault, and B. S. Hart, 2009, A visual data mining methodology to conduct seismic facies analysis: Part 2—Application to 3D seismic data: Geophysics, v. 74, p. P1–P11.
- ↑ Parmelin, H., 1963, Picasso plain: An intimate portrait: London, Secker & Warburg, 250 p., quotation on p. 42
- ↑ 295.0 295.1 Neal, J., and V. Abreu, 2009, Sequence stratigraphy hierarchy and the accommodation succession method: Geology, v. 37, p. 779–782.
- ↑ 296.0 296.1 Abreu, V., K. Pederson, J. Neal, and K. M. Bohacs, 2014, A simplified guide for sequence stratigraphy: Nomenclature, definitions and method: Geological Society of America Annual Meeting, 19–22 October 2014, Vancouver, British Columbia, Abstracts with Programs, v. 46, no. 6, p. 832.
- ↑ Schwalbach, J. R., and K. M. Bohacs, 1995, Stratigraphic sections and gamma-ray spectrometry from five outcrops of the Monterey Formation in southwestern California; Naples Beach, Point Pedernales, Lion’s Head, Shell Beach, and Point Buchon: US Geological Survey Bulletin 1995, p. Q1–Q39.
- ↑ Kahn, A. E., 1970, Joys and sorrows: Reflections by Pablo Casals: New York, Simon & Schuster, 314 p., quotation p. 224
- ↑ Grabau, A. W., 1913, Principles of stratigraphy: New York, A. G. Seiler, 1185 p.
- ↑ Barrell, J., 1917, Rhythms and the measurements of geological time: Geological Society of America Bulletin, v. 28, p. 745–904.
- ↑ Sloss, L. L., W. C. Krumbein, and E. C. Dapples, 1949, Integrated facies analysis, in C. R. Longwell, ed., Sedimentary facies in geologic history: Geological Society of America Memoir 39, p. 91–124.
- ↑ Wheeler, H. E., and H. H. Murray, 1957, Base level control patterns in cyclothemic sedimentation: AAPG Bulletin, v. 41, p. 1985–2011.
- ↑ Wheeler, H. E., 1958. Time stratigraphy: AAPG Bulletin, v. 42, p. 1047–1063.
- ↑ Wheeler, H. E., 1959, Unconformity bounded units in stratigraphy: AAPG Bulletin, v. 43, p. 1975–1977.
- ↑ 305.0 305.1 Sloss, L. L., 1962, Stratigraphic models in exploration: AAPG Bulletin, v. 46, p. 1050–1057.
- ↑ 306.0 306.1 Sloss, L. L., 1963, Sequence in the cratonic interior of North America: Geological Society of America Bulletin, v. 74, p. 93–114.
- ↑ Curray, J. R., 1964, Transgressions and regressions, in R. L. Miller, ed., Papers in marine geology: Shepard commemorative volume: New York, Macmillan, p. 175–203.
- ↑ Frazier, D. E., 1974, Depositional episodes: Their relationship to the Quaternary stratigraphic framework in the north-western portion of the Gulf Basin: Geological Circular, v. 1, p. 28.
- ↑ 309.0 309.1 309.2 Vail, P. R., 1975, Eustatic cycles from seismic data for global stratigraphic analysis (abstract): AAPG Bulletin, v. 59, p. 2198–2199.
- ↑ 310.0 310.1 310.2 310.3 Vail, P. R., R. M. Mitchum Jr., and S. Thompson III, 1977a, Seismic stratigraphy and global changes of sea level, Part 3: Relative changes of sea level from coastal onlap, in C. E. Payton, ed., Seismic stratigraphy—applications to hydrocarbon exploration: AAPG Memoir 26, p. 63–81.
- ↑ 311.0 311.1 311.2 Vail, P. R., R. M. Mitchum Jr., and S. Thompson III, 1977b, Seismic stratigraphy and global changes of sea level. Part 4: Global cycles of relative changes of sea level, in C. E. Payton, ed., Seismic stratigraphy—applications to hydrocarbon exploration: AAPG Memoir 26, p. 83–97.
- ↑ 312.0 312.1 312.2 Vail, P. R., R. G. Todd, and J. B. Sangree, 1977c, Seismic stratigraphy and global changes of sea level, Part 5: Chronostratigraphic significance of seismic reflections, in C. E. Payton, eds., Seismic stratigraphy—applications to hydrocarbon exploration: AAPG Memoir 26, p. 99–116.
- ↑ 313.0 313.1 313.2 Vail, P. R., F. Audemard, S. A. Bowman, P. N. Eisner, and C. Perez-Cruz, 1991, The stratigraphic signatures of tectonics, eustasy and sedimentology-an overview, in G. Einsele, W. Ricken, and A. Seilacher, eds., Cycles and events in stratigraphy: Berlin, Springer-Verlag, p. 617–659.
- ↑ Bohacs, K. M., 1990, Sequence stratigraphy of the Monterey Formation, Santa Barbara County: Integration of physical, chemical, and biofacies data from outcrop and subsurface, in M. M. Keller, and M. K. McGowen, eds., Miocene and Oligocene petroleum reservoirs of the Santa Maria and Santa Barbara-Ventura basins, California: SEPM Core Workshop 14, p. 139–201.
- ↑ Bohacs, K. M., 1993, Source quality variations tied to sequence development in the Monterey and associated formations, southwestern California, in B. J. Katz, and L. M. Pratt, eds., Petroleum source rocks in a sequence-stratigraphic framework: AAPG Studies in Geology 7, p. 177–204.
- ↑ 316.0 316.1 316.2 Van Wagoner, J. C., R. M. Mitchum Jr., K. M. Campion, and V. D. Rahmanian, 1990, Siliciclastic sequence stratigraphy in well logs, core, and outcrops: Concepts for high-resolution correlation of time and facies: AAPG Methods in Exploration Series 7, 55 p.
- ↑ Posamentier, H. W., G. P. Allen, and D. P. James, 1992, High resolution sequence stratigraphy-The east Coulee Delta, Alberta: Journal of Sedimentary Petrology, v. 62, p. 310–317.
- ↑ Van Wagoner, J. C., and G. T. Bertram, 1995, Sequence stratigraphy of foreland basin deposits: AAPG Memoir 64, 487 p.
- ↑ Posamentier, H. W., and G. P. Allen, 1999, Siliciclastic sequence stratigraphy—Concepts and applications: SEPM Concepts in Sedimentology and Paleontology, v. 7, p. 216.
- ↑ Adams, L. K., J. H. S. Macquaker, and J. D. Marshall, 2006, Iron(III)-reduction in a low-organic-carbon brackish-marine system: Journal of Sedimentary Research, v. 76, p. 919–925.
- ↑ Loutit, T. S., J. Hardenbol, P. R. Vail, and G. R. Baum, 1988, Condensed sections: The key to age dating and correlation of continental margin sequences, in C. K. Wilgus, B. S. Hastings, C. G. Kendall, H. W. Posamentier, C. A. Ross, and J. C. Van Wagoner, eds., Sea-level changes: An integrated approach: SEPM Special Publication 42, p. 183–213
- ↑ Schieber, J., 1990, Significance of styles of epicontinental; shale sedimentation in the Belt Basin, mid-Proterozoic of Montana, USA: Sedimentary Geology, v. 69, p. 297–312.
- ↑ Wignall, P. B., and J. R. Maynard, 1993, The sequence stratigraphy of transgressive black shales, in B. J. Katz, and L. Pratt, eds., Source rocks within a sequence stratigraphic framework: AAPG Studies in Geology 37, p. 35–47.
- ↑ Witzke, B. J., G. A. Ludvigson, and J. Day, 1996, Introduction: Paleozoic applications of sequence stratigraphy, in B. J. Witzke, G. A. Ludvigson, and J. Day, eds., Paleozoic sequence stratigraphy: Views from the North American Craton: Geological Society of America Special Paper 306, p. 1–6.
- ↑ Bohacs, K. M., and J. Suter, 1997, Sequence stratigraphic distribution of coaly rocks: Fundamental controls and paralic examples: AAPG Bulletin, v. 81, p. 1612–1639.
- ↑ Bohacs, K. M., A. R. Carroll, and J. E. Neal, 2003, Lessons from large lake systems—thresholds, nonlinearity, and strange attractors, in M. A. Chan, and A. W. Archer, eds., Extreme depositional environments: Mega end members in geologic time: Geological Society of America Special Paper 370, p. 75–90.
- ↑ 327.0 327.1 Bohacs, K. M., G. J. Grabowski Jr., A. R. Carroll, K. J. Miskell-Gerhardt, and K. Glaser, 2007, Lithofacies architecture and sequence stratigraphy of the Green River Formation, greater Green River Basin, Wyoming and Colorado: Mountain Geologist, v. 44, p. 39–60.
- ↑ Macquaker, J. H. S., and C. R. Jones, 2002, A sequence-stratigraphic study of mudstone heterogeneity: A combined petrographic/wireline log investigation of Upper Jurassic mudstones from the North Sea (U.K.), in M. Lowell, and N. Parkinson, eds., Geological applications of well logs: AAPG Methods in Exploration 13, p. 123–141.
- ↑ Brett, C. E., G. C. Baird, and A. J. Bartholomew, 2004, Sequence stratigraphy of highly variable Middle Devonian strata in Central Kentucky: Implications for regional correlations and depositional environments, in J. Schieber, and O. R. Lazar, eds., Devonian black shales of the Eastern US: New insights into sedimentology and stratigraphy from the subsurface and outcrops in the Illinois and Appalachian basins: Indiana Geological Survey Open File Study 04-05, p. 35–60.
- ↑ 330.0 330.1 330.2 330.3 Mitchum Jr., R. M., 1977, Seismic stratigraphy and global changes of sea level, Part 11: Glossary of terms used in seismic stratigraphy, in C. E. Payton, ed., Seismic stratigraphy—Applications to hydrocarbon exploration: AAPG Memoir 26, p. 205–212.
- ↑ 331.0 331.1 Posamentier, H. W., M. T. Jervey, and P. R. Vail, 1988, Eustatic controls on clastic deposition. I. Conceptual framework, in C. K. Wilgus, B. S. Hastings, C. G. St. C. Kendall, H. W. Posamentier, C. A. Ross, and J. C. Van Wagoner, eds., Sea level changes-an integrated approach: SEPM Special Publication 42, p. 110–124.
- ↑ 332.0 332.1 332.2 Van Wagoner, J. C., H. W. Posamentier, R. M. Mitchum, P. R. Vail, P. R., J. F. Sarg, T. S. Loutit, and J. Hardenbol, 1988, An overview of sequence stratigraphy and key definitions, in C. K. Wilgus, B. S. Hastings, C. G. St. C. Kendall, H. W. Posamentier, C. A. Ross, and J. C. Van Wagoner, eds., Sea level changes—an integrated approach: SEPM Special Publication 42, p. 39–45.
- ↑ Steno, N., 1667, Canis carchariae dissectum caput, in V. Maar, ed., Nicolai Stenonis Opera Philosophica: Copenhagen, Denmark, Christian Christensen, (1910), 264 p.
- ↑ Smith, W., 1815, A Geological Map of England and Wales and Part of Scotland. (2006 reprint, London, British Geological Survey)
- ↑ Lyell, C., 1830, Principles of geology, vol. 1: London, J. Murray, 511 p.
- ↑ Lyell, C., 1835, The Bakerian lecture—On the proofs of a gradual rising of the land in certain parts of Sweden: Philosophical Transactions of the Royal Society of London, v. 125, p. 1–38.
- ↑ Walther, J., 1894, Einleitung in die Geologie als Historische Wissenschaft: Fischer Verlag, Jena, p. 535–1055.
- ↑ Chamberlain, T. C., 1898, The ulterior basis of time divisions and the classification of geologic history: Journal of Geology, v. 6, p. 449–462.
- ↑ Chamberlain, T. C., 1909, Diastrophism as the ultimate basis of correlation: Journal of Geology, v. 17, p. 685–693.
- ↑ Van Siclen, D. C., 1958, Depositional topography—examples and theory: AAPG Bulletin v. 42, p. 1897–1913.
- ↑ Guthrie, J. M., and K. M. Bohacs, 2009, Spatial variability of organic-matter-rich rocks: A critical element for defining the petroleum system of Pennsylvanian carbonate reservoirs of the paradox basin, SE Utah, in W. S. Houston, L. L. Wray, and P. G. Moreland, eds., The paradox basin revisited—new developments in petroleum systems and basin analysis: RMAG Special Publication 2009, p. 95–130.
- ↑ Paola, C., P. L. Heller, P. L., and C. L. Angevine, 1992, The large-scale dynamics of grain-size variation in alluvial basins, 1: Theory: Basin Research, v. 4, p. 73–90.
- ↑ 343.0 343.1 343.2 343.3 343.4 343.5 Bohacs, K. M., G. J. Grabowski Jr., and J. E. Neal, 2004, Unlocking geological history: The key roles of mudstones and sequence stratigraphy, in J. Schieber, and O. R. Lazar, eds., Devonian black shales of the Eastern US: New insights into sedimentology and stratigraphy from the subsurface and outcrops in the Illinois and Appalachian basins: Indiana Geological Survey Open File Study 04-05, p. 78.
- ↑ 344.0 344.1 Bohacs, K. M., and O. R. Lazar, 2008, The role of sequence stratigraphy in unraveling and applying the complex controls from mudstone reservoir properties: AAPG Search and Discovery article #90078.
- ↑ Bohacs, K. M., and O. R. Lazar, 2010, Sequence stratigraphy in fine-grained rocks, in J. Schieber, O. R. Lazar, and K. M. Bohacs, eds., Sedimentology and stratigraphy of shales: Expression and correlation of depositional sequences in the Devonian of Tennessee, Kentucky, and Indiana: SEPM Field Trip Guidebook, p. 15–30.
- ↑ Bohacs, K., and R. Lazar, 2022, The importance of and challenges in studying fine-grained sedimentary rocks—Introduction and overview, in K. M. Bohacs and O. R. Lazar, eds., Sequence stratigraphy: Applications to fine-grained rocks: AAPG Memoir 126, p. 1–20.